IEEE Account
- Change Username/Password
- Update Address

Purchase Details
- Payment Options
- Order History
- View Purchased Documents
Profile Information
- Communications Preferences
- Profession and Education
- Technical Interests
- US & Canada: +1 800 678 4333
- Worldwide: +1 732 981 0060
- Contact & Support
- About IEEE Xplore
- Accessibility
- Terms of Use
- Nondiscrimination Policy
- Privacy & Opting Out of Cookies
A not-for-profit organization, IEEE is the world's largest technical professional organization dedicated to advancing technology for the benefit of humanity. © Copyright 2024 IEEE - All rights reserved. Use of this web site signifies your agreement to the terms and conditions.
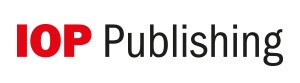
We apologize for the inconvenience...
To ensure we keep this website safe, please can you confirm you are a human by ticking the box below.
If you are unable to complete the above request please contact us using the below link, providing a screenshot of your experience.
https://ioppublishing.org/contacts/
Thank you for visiting nature.com. You are using a browser version with limited support for CSS. To obtain the best experience, we recommend you use a more up to date browser (or turn off compatibility mode in Internet Explorer). In the meantime, to ensure continued support, we are displaying the site without styles and JavaScript.
- View all journals
- Explore content
- About the journal
- Publish with us
- Sign up for alerts
- Published: 03 August 2020
Impacts of climate change on energy systems in global and regional scenarios
- Seleshi G. Yalew ORCID: orcid.org/0000-0002-7304-6750 1 , 2 , 3 ,
- Michelle T. H. van Vliet 2 , 4 ,
- David E. H. J. Gernaat ORCID: orcid.org/0000-0003-4994-1453 1 , 5 ,
- Fulco Ludwig 2 ,
- Ariel Miara ORCID: orcid.org/0000-0001-7089-4765 6 , 7 ,
- Chan Park ORCID: orcid.org/0000-0002-4994-6855 8 ,
- Edward Byers ORCID: orcid.org/0000-0003-0349-5742 9 ,
- Enrica De Cian 10 , 11 ,
- Franziska Piontek 12 ,
- Gokul Iyer ORCID: orcid.org/0000-0002-3565-7526 13 ,
- Ioanna Mouratiadou ORCID: orcid.org/0000-0002-3541-6271 1 ,
- James Glynn ORCID: orcid.org/0000-0001-7004-0153 14 ,
- Mohamad Hejazi 13 ,
- Olivier Dessens 15 ,
- Pedro Rochedo ORCID: orcid.org/0000-0001-5151-0893 16 ,
- Robert Pietzcker ORCID: orcid.org/0000-0002-9403-6711 12 ,
- Roberto Schaeffer ORCID: orcid.org/0000-0002-3709-7323 16 ,
- Shinichiro Fujimori ORCID: orcid.org/0000-0001-7897-1796 17 , 18 ,
- Shouro Dasgupta ORCID: orcid.org/0000-0003-4080-8066 10 , 11 ,
- Silvana Mima 19 ,
- Silvia R. Santos da Silva ORCID: orcid.org/0000-0002-6493-1475 13 , 20 ,
- Vaibhav Chaturvedi 21 ,
- Robert Vautard ORCID: orcid.org/0000-0001-5544-9903 22 &
- Detlef P. van Vuuren ORCID: orcid.org/0000-0003-0398-2831 1 , 5
Nature Energy volume 5 , pages 794–802 ( 2020 ) Cite this article
13k Accesses
206 Citations
128 Altmetric
Metrics details
- Projection and prediction
Although our knowledge of climate change impacts on energy systems has increased substantially over the past few decades, there remains a lack of comprehensive overview of impacts across spatial scales. Here, we analyse results of 220 studies projecting climate impacts on energy systems globally and at the regional scale. Globally, a potential increase in cooling demand and decrease in heating demand can be anticipated, in contrast to slight decreases in hydropower and thermal energy capacity. Impacts at the regional scale are more mixed and relatively uncertain across regions, but strongest impacts are reported for South Asia and Latin America. Our assessment shows that climate impacts on energy systems at regional and global scales are uncertain due partly to the wide range of methods and non-harmonized datasets used. For a comprehensive assessment of climate impacts on energy, we propose a consistent multi-model assessment framework to support regional-to-global-scale energy planning.
This is a preview of subscription content, access via your institution
Access options
Access Nature and 54 other Nature Portfolio journals
Get Nature+, our best-value online-access subscription
24,99 € / 30 days
cancel any time
Subscribe to this journal
Receive 12 digital issues and online access to articles
111,21 € per year
only 9,27 € per issue
Buy this article
- Purchase on SpringerLink
- Instant access to full article PDF
Prices may be subject to local taxes which are calculated during checkout
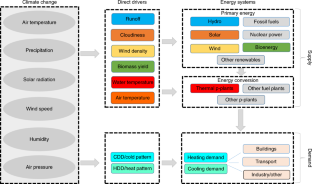
Similar content being viewed by others
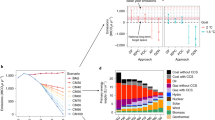
A framework for national scenarios with varying emission reductions
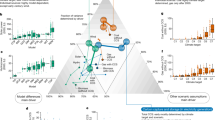
Spread in climate policy scenarios unravelled
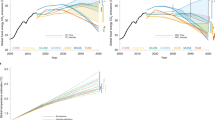
A multi-model analysis of long-term emissions and warming implications of current mitigation efforts
Data availability.
All data that support the findings of this study presented in the figures are provided in the Source Data section associated with this manuscript. Source data are provided with this paper.
Bruckner, T. et al. in Climate Change 2014: Mitigation of Climate Change (eds Edenhofer, O. et al.) (Cambridge Univ. Press, 2014).
Schaeffer, R. et al. Energy sector vulnerability to climate change: a review. Energy 38 , 1–12 (2012).
Google Scholar
Ebinger, J. & Vergara, W. Climate Impacts on Energy Systems: Key Issues for Energy Sector Adaptation (The World Bank, 2011).
Crook, J. A., Jones, L. A., Forster, P. M. & Crook, R. Climate change impacts on future photovoltaic and concentrated solar power energy output. Energ. Environ, Sci. 4 , 3101–3109 (2011).
Owusu, P. A. & Asumadu-Sarkodie, S. A review of renewable energy sources, sustainability issues and climate change mitigation. Cogent. Eng. 3 , 1167990 (2016).
Bartos, M. D. & Chester, M. V. Impacts of climate change on electric power supply in the Western United States. Nat. Clim. Change 5 , 748–752 (2015).
Karnauskas, K. B., Lundquist, J. K. & Zhang, L. Southward shift of the global wind energy resource under high carbon dioxide emissions. Nar. Geosci. 11 , 38–43 (2018).
Craig, M. T. et al. A review of the potential impacts of climate change on bulk power system planning and operations in the United States. Renew. Sust. Energ. Rev. 98 , 255–267 (2018).
Van Vliet, M. T. et al. Vulnerability of US and European electricity supply to climate change. Nat. Clim. Change 2 , 676 (2012).
Liu, L., Hejazi, M., Li, H., Forman, B. & Zhang, X. Vulnerability of US thermoelectric power generation to climate change when incorporating state-level environmental regulations. Nat. Ener. 2 , 17109 (2017).
Ciscar, J.-C. & Dowling, P. Integrated assessment of climate impacts and adaptation in the energy sector. Energy Econ. 46 , 531–538 (2014).
Ravestein, P., van der Schrier, G., Haarsma, R., Scheele, R. & van den Broek, M. Vulnerability of European intermittent renewable energy supply to climate change and climate variability. Renew. Sust. Ener. Rev. 97 , 497–508 (2018).
Perera, A., Nik, V. M., Chen, D., Scartezzini, J. L. & Hong, T. Quantifying the impacts of climate change and extreme climate events on energy systems. Nat. Energy 5 , 150–159 (2020).
IPCC Climate Change 2014: Synthesis Report (eds Core Writing Team, Pachauri, R. K. & Meyer L. A.) (IPCC, 2014).
Isaac, M. & van Vuuren, D. P. Modeling global residential sector energy demand for heating and air conditioning in the context of climate change. Energy Policy 37 , 507–521 (2009).
IPCC Climate Change 2014: Impacts, Adaptation, and Vulnerability (eds Field, C. B. et al.) (Cambridge Univ. Press, 2014).
Cinner, J. E. et al. Building adaptive capacity to climate change in tropical coastal communities. Nat. Clim. Change 8 , 177–123 (2018).
Lumbroso, D., Woolhouse, G. & Jones, L. A review of the consideration of climate change in the planning of hydropower schemes in sub-Saharan Africa. Clim. Change 133 , 621–633 (2015).
Kabir, E., Kumar, P., Kumar, S., Adelodun, A. A. & Kim, K.-H. Solar energy: potential and future prospects. Renew. Sust. Energ. Rev. 82 , 894–900 (2018).
Pryor, S. & Barthelmie, R. Climate change impacts on wind energy: a review. Renew. Sust. Energ. Rev. 14 , 430–437 (2010).
Berndes, G., Hoogwijk, M. & Van den Broek, R. The contribution of biomass in the future global energy supply: a review of 17 studies. Biomass Bioenerg. 25 , 1–28 (2003).
Li, D. H., Yang, L. & Lam, J. C. Impact of climate change on energy use in the built environment in different climate zones–a review. Energy 42 , 103–112 (2012).
Auffhammer, M. & Mansur, E. T. Measuring climatic impacts on energy consumption: a review of the empirical literature. Energy Econ. 46 , 522–530 (2014).
Mideksa, T. K. & Kallbekken, S. The impact of climate change on the electricity market: a review. Energy Policy 38 , 3579–3585 (2010).
Chandramowli, S. N. & Felder, F. A. Impact of climate change on electricity systems and markets–a review of models and forecasts. Sust. Energ. Technol. Assess. 5 , 62–74 (2014).
Mikellidou, C. V., Shakou, L. M., Boustras, G. & Dimopoulos, C. Energy critical infrastructures at risk from climate change: a state of the art review. Safety Science 110 , https://doi.org/10.1016/j.ssci.2017.12.022 (2017).
Stanton, M. C. B., Dessai, S. & Paavola, J. A systematic review of the impacts of climate variability and change on electricity systems in Europe. Energy 109 , 1148–1159 (2016).
Cronin, J., Anandarajah, G. & Dessens, O. Climate change impacts on the energy system: a review of trends and gaps. Clim. Change 151 , 79–93 (2018).
Barnett, T. P., Adam, J. C. & Lettenmaier, D. P. Potential impacts of a warming climate on water availability in snow-dominated regions. Nature 438 , 303 (2005).
Chilkoti, V., Bolisetti, T. & Balachandar, R. Climate change impact assessment on hydropower generation using multi-model climate ensemble. Renew. Energ. 109 , 510–517 (2017).
Fan, J.-L. Impacts of climate change on hydropower generation in China. Comput. Simulat. 167 , 4–18 (2018).
MathSciNet Google Scholar
Teotónio, C., Fortes, P., Roebeling, P., Rodriguez, M. & Robaina-Alves, M. Assessing the impacts of climate change on hydropower generation and the power sector in Portugal: a partial equilibrium approach. Renew. Sust. Energ. Rev. 74 , 788–799 (2017).
Hamududu, B. & Killingtveit, A. Assessing climate change impacts on global hydropower. Energies 5 , 305–322 (2012).
Van Vliet, M. T., Wiberg, D., Leduc, S. & Riahi, K. Power-generation system vulnerability and adaptation to changes in climate and water resources. Nat. Clim. Change 6 , 375–380 (2016).
Van Vliet, M. et al. Multi-model assessment of global hydropower and cooling water discharge potential under climate change. Global Environ. Change 40 , 156–170 (2016).
Turner, S. W., Ng, J. Y. & Galelli, S. Examining global electricity supply vulnerability to climate change using a high-fidelity hydropower dam model. Sci. Total Environ. 590 , 663–675 (2017).
Zhou, Y. et al. A comprehensive view of global potential for hydro-generated electricity. Energ. Environ. Sci. 8 , 2622–2633 (2015).
Raje, D. & Mujumdar, P. Reservoir performance under uncertainty in hydrologic impacts of climate change. Adv. Water Resour. 33 , 312–326 (2010).
Gaudard, L., Gilli, M. & Romerio, F. Climate change impacts on hydropower management. Water Resource. Manag. 27 , 5143–5156 (2013).
Mohor, G. S., Rodriguez, D. A., Tomasella, J. & Júnior, J. L. S. Exploratory analyses for the assessment of climate change impacts on the energy production in an Amazon run-of-river hydropower plant. J. Hydrol. Reg. Studies 4 , 41–59 (2015).
Fant, C., Schlosser, C. A. & Strzepek, K. The impact of climate change on wind and solar resources in southern Africa. Appl. Energy 161 , 556–564 (2016).
Wachsmuth, J. et al. How will renewable power generation be affected by climate change? The case of a Metropolitan Region in Northwest Germany. Energy 58 , 192–201 (2013).
Jerez, S. et al. The impact of climate change on photovoltaic power generation in Europe. Nat. Commun. 6 , 10014 (2015).
Bartók, B. et al. Projected changes in surface solar radiation in CMIP5 global climate models and in EURO-CORDEX regional climate models for Europe. Climate Dynam. 49 , 2665–2683 (2017).
Davy, R., Gnatiuk, N., Pettersson, L. & Bobylev, L. Climate change impacts on wind energy potential in the European domain with a focus on the Black Sea. Renew. Sust. Energ. Rev. 81 , 1652–1659 (2017).
Carvalho, D., Rocha, A., Gómez-Gesteira, M. & Santos, C. S. Potential impacts of climate change on European wind energy resource under the CMIP5 future climate projections. Renew. Energ. 101 , 29–40 (2017).
Hueging, H., Haas, R., Born, K., Jacob, D. & Pinto, J. G. Regional changes in wind energy potential over Europe using regional climate model ensemble projections. J. Appl. Meteorol. Climatol. 52 , 903–917 (2013).
Tobin, I. et al. Vulnerabilities and resilience of European power generation to 1.5 °C, 2 °C and 3 °C warming. Environ. Res. Lett. 13 , 044024 (2018).
Vautard, R. et al. Regional climate model simulations indicate limited climatic impacts by operational and planned European wind farms. Nat. Commun. 5 , 3196 (2014).
Jerez, S. et al. Future changes, or lack thereof, in the temporal variability of the combined wind-plus-solar power production in Europe. Renew. Energy 139 , 251–260 (2019).
De Lucena, A. F. P., Szklo, A. S., Schaeffer, R. & Dutra, R. M. The vulnerability of wind power to climate change in Brazil. Renew. Energy 35 , 904–912 (2010).
Pereira, E. B., Martins, F. R., Pes, M. P., da Cruz Segundo, E. I. & Lyra, Ad. A. The impacts of global climate changes on the wind power density in Brazil. Renew. Energy 49 , 107–110 (2013).
Breslow, P. B. & Sailor, D. J. Vulnerability of wind power resources to climate change in the continental United States. Renew. Energy 27 , 585–598 (2002).
Sailor, D. J., Smith, M. & Hart, M. Climate change implications for wind power resources in the Northwest United States. Renew. Energy 33 , 2393–2406 (2008).
De Jong, P. et al. Estimating the impact of climate change on wind and solar energy in Brazil using a South American regional climate model. Renew. Energy 141 , 390–401 (2019).
Tuck, G., Glendining, M. J., Smith, P., House, J. I. & Wattenbach, M. The potential distribution of bioenergy crops in Europe under present and future climate. Biomass Bioenerg. 30 , 183–197 (2006).
Bellarby, J., Wattenbach, M., Tuck, G., Glendining, M. J. & Smith, P. The potential distribution of bioenergy crops in the UK under present and future climate. Biomass Bioenerg. 34 , 1935–1945 (2010).
Harvey, M. & Pilgrim, S. The new competition for land: food, energy, and climate change. Food Policy 36 , S40–S51 (2011).
Kyle, P., Müller, C., Calvin, K. & Thomson, A. Meeting the radiative forcing targets of the representative concentration pathways in a world with agricultural climate impacts. Earth’s Future 2 , 83–98 (2014).
Miara, A., Vörösmarty, C. J., Stewart, R. J., Wollheim, W. M. & Rosenzweig, B. Riverine ecosystem services and the thermoelectric sector: strategic issues facing the Northeastern United States. Environ. Res. Lett. 8 , 025017 (2013).
Miara, A. et al. Climate and water resource change impacts and adaptation potential for US power supply. Nat. Clim. Change 7 , 793–798 (2017).
Miara, A. et al. Climate-water adaptation for future US electricity infrastructure. Environ. Sci. Technol. 53 , 14029–14040 (2019).
Byers, E., Hall, J., Amezaga, J., O’Donnell, G. & Leathard, A. Water and climate risks to power generation with carbon capture and storage. Environ. Res. Lett. 11 , 024011 (2016).
Angeles, M. E., González, J. E. & Ramírez, N. Impacts of climate change on building energy demands in the intra-Americas region. Theoret. Appl. Climatol 133 , 59–72 (2018).
Fan, J.-L., Hu, J.-W. & Zhang, X. Impacts of climate change on electricity demand in China: an empirical estimation based on panel data. Energy 170 , 880–888 (2019).
Taseska, V., Markovska, N. & Callaway, J. M. Evaluation of climate change impacts on energy demand. Energy 48 , 88–95 (2012).
Allen, M. R., Fernandez, S. J., Fu, J. S. & Olama, M. M. Impacts of climate change on sub-regional electricity demand and distribution in the southern United States. Nat. Energy 1 , 16103 (2016).
Zhou, Y. et al. Modeling the effect of climate change on US state-level buildings energy demands in an integrated assessment framework. Appl. Energy 113 , 1077–1088 (2014).
Hadley, S. W., Erickson Iii, D. J., Hernandez, J. L., Broniak, C. T. & Blasing, T. J. Responses of energy use to climate change: a climate modeling study. Geophys. Res. Lett. 33 , https://doi.org/10.1029/2006GL026652 (2006).
Eom, J., Clarke, L., Kim, S. H., Kyle, P. & Patel, P. China’s building energy demand: long-term implications from a detailed assessment. Energy 46 , 405–419 (2012).
McFarland, J. et al. Impacts of rising air temperatures and emissions mitigation on electricity demand and supply in the United States: a multi-model comparison. Clim. Change 131 , 111–125 (2015).
Clarke, L. et al. Effects of long-term climate change on global building energy expenditures. Energy Econ. 72 , 667–677 (2018).
Labriet, M. et al. Worldwide impacts of climate change on energy for heating and cooling. Mit. Adapt. Strat. Global Change 20 , 1111–1136 (2015).
Van Ruijven, B. J., De Cian, E. & Wing, I. S. Amplification of future energy demand growth due to climate change. Nat. Commun. 10 , 2762 (2019).
De Cian, E. & Wing, I. S. Global energy consumption in a warming climate. Environ. Res. Econ. 72 , 365–410 (2019).
Auffhammer, M., Baylis, P. & Hausman, C. H. Climate change is projected to have severe impacts on the frequency and intensity of peak electricity demand across the United States. Proc. Natl Acad. Sci. USA 114 , 1886–1891 (2017).
De Cian, E., Lanzi, E. & Roson, R. Seasonal temperature variations and energy demand. Clim. Change 116 , 805–825 (2013).
Invidiata, A. & Ghisi, E. Impact of climate change on heating and cooling energy demand in houses in Brazil. Energ. Buildings 130 , 20–32 (2016).
Wang, H. & Chen, Q. Impact of climate change heating and cooling energy use in buildings in the United States. Energ. Buildings 82 , 428–436 (2014).
Hamlet, A. F., Lee, S.-Y., Mickelson, K. E. & Elsner, M. M. Effects of projected climate change on energy supply and demand in the Pacific Northwest and Washington State. Clim. Change 102 , 103–128 (2010).
Davis, L. W. & Gertler, P. J. Contribution of air conditioning adoption to future energy use under global warming. Proc. Natl Acad. Sci. USA 112 , 5962–5967 (2015).
Park, C. et al. Avoided economic impacts of energy demand changes by 1.5 and 2 °C climate stabilization. Environ. Res. Lett. 13 , 045010 (2018).
Waite, M. et al. Global trends in urban electricity demands for cooling and heating. Energy 127 , 786–802 (2017).
Morakinyo, T. E. et al. Estimates of the impact of extreme heat events on cooling energy demand in Hong Kong. Renew. Energy 142 , 73–84 (2019).
Moazami, A., Nik, V. M., Carlucci, S. & Geving, S. Impacts of future weather data typology on building energy performance–Investigating long-term patterns of climate change and extreme weather conditions. Appl. Energy 238 , 696–720 (2019).
Dirks, J. A. et al. Impacts of climate change on energy consumption and peak demand in buildings: a detailed regional approach. Energy 79 , 20–32 (2015).
D’Oca, S., Hong, T. & Langevin, J. The human dimensions of energy use in buildings: a review. Renew. Sust. Energy Rev. 81 , 731–742 (2018).
Poortinga, W., Steg, L. & Vlek, C. Values, environmental concern, and environmental behavior: a study into household energy use. Environ. Behav. 36 , 70–93 (2004).
De Cian, E., Pavanello, F., Randazzo, T., Mistry, M. N. & Davide, M. Households’ adaptation in a warming climate. Air conditioning and thermal insulation choices. Environ. Sci. Policy 100 , 136–157 (2019).
Castleton, H. F., Stovin, V., Beck, S. B. & Davison, J. B. Green roofs; building energy savings and the potential for retrofit. Energy Buildings 42 , 1582–1591 (2010).
Jones, P., Lannon, S. & Patterson, J. Retrofitting existing housing: how far, how much? Building Res. Info. 41 , 532–550 (2013).
Da Silva Soito, J. L. & Freitas, M. A. V. J. R. Amazon and the expansion of hydropower in Brazil: vulnerability, impacts and possibilities for adaptation to global climate change. Renew. Sust. Energy Rev. 15 , 3165–3177 (2011).
Cohen, S. M., Macknick, J., Averyt, K. & Meldrum, J. Modeling Climate-Water Impacts on Electricity Sector Capacity Expansion (National Renewable Energy Laboratory, 2014).
Mima, S. & Criqui, P. The costs of climate change for the European energy system, an assessment with the POLES model. Environ. Model. Ass. 20 , 303–319 (2015).
Ackerman, F. & Stanton, E. A. The Cost of Climate Change: What We’ll Pay if Global Warming Continues Unchecked (Natural Resources Defence Council, 2008).
Turner, S. W., Hejazi, M., Kim, S. H., Clarke, L. & Edmonds, J. Climate impacts on hydropower and consequences for global electricity supply investment needs. Energy 141 , 2081–2090 (2017).
Van der Linden, P. & Mitchell, J. (eds) ENSEMBLES: Climate Change and its Impacts—Summary of Research and Results from the ENSEMBLES Project (European Environment Agency, 2009).
Rübbelke, D. & Vögele, S. Impacts of climate change on European critical infrastructures: the case of the power sector. Environ. Sci. Policy 14 , 53–63 (2011).
Pryor, S. & Barthelmie, R. Assessing the vulnerability of wind energy to climate change and extreme events. Clim. Change 121 , 79–91 (2013).
Miller, N. L., Hayhoe, K., Jin, J. & Auffhammer, M. Climate, extreme heat, and electricity demand in California. J. Appl. Meteorol. Climatol. 47 , 1834–1844 (2008).
Forzieri, G. et al. Escalating impacts of climate extremes on critical infrastructures in Europe. Global Environ. Change 48 , 97–107 (2018).
Bartos, M. et al. Impacts of rising air temperatures on electric transmission ampacity and peak electricity load in the United States. Environ. Res. Lett. 11 , 114008 (2016).
Panteli, M. & Mancarella, P. Influence of extreme weather and climate change on the resilience of power systems: Impacts and possible mitigation strategies. Electric Power Syst. Res. 127 , 259–270 (2015).
Dowling, P. The impact of climate change on the European energy system. Energy Policy 60 , 406–417 (2013).
Haddeland, I. et al. Multimodel estimate of the global terrestrial water balance: setup and first results. J. Hydrometeorol. 12 , 869–884 (2011).
Schewe, J. et al. Multimodel assessment of water scarcity under climate change. Proc. Natl Acad. Sci. USA 111 , 3245–3250 (2014).
Rosenzweig, C. et al. The agricultural model intercomparison and improvement project (AgMIP): protocols and pilot studies. Agri. Forest Meteorol. 170 , 166–182 (2013).
van Vuuren, D. P. & Carter, T. R. Climate and socio-economic scenarios for climate change research and assessment: reconciling the new with the old. Clim. Change 122 , 415–429 (2014).
Van Vuuren, D. P. et al. A new scenario framework for climate change research: scenario matrix architecture. Clim. Change 122 , 373–386 (2014).
O’Neill, B. C. et al. A new scenario framework for climate change research: the concept of shared socioeconomic pathways. Clim. Change 122 , 387–400 (2014).
Wiedenhofer, D., Lenzen, M. & Steinberger, J. K. Energy requirements of consumption: urban form, climatic and socio-economic factors, rebounds and their policy implications. Energy Policy 63 , 696–707 (2013).
Frieler, K. et al. Assessing the impacts of 1.5 C global warming–simulation protocol of the Inter-Sectoral Impact Model Intercomparison Project (ISIMIP2b). Geosci. Model Dev. 10 , 4321–4345 (2017).
Download references
Acknowledgements
We wish to thank the JPI Climate initiative and participating grant institutes for funding the ISIpedia project. We also thank J. Burrough for professional advice on the English of a near-final draft. E.d.C. has received funding from the European Research Council (ERC) under the European Union’s Horizon 2020 research and innovation programme under grant agreement no. 756194 (ENERGYA). J.G. is supported by a research grant from Science Foundation Ireland (SFI) and the National Natural Science Foundation of China (NSFC) under the SFI-NSFC Partnership Programme, grant no. 17/NSFC/5181. D.P.v.V., R.S. and D.E.H.J.G. are supported by the Horizon 2020 NAVIGATE project, and D.P.v.V., R.S. and D.E.H.J.G. also acknowledge support from the COMMIT and Horizon 2020 ENGAGE project. F.P. acknowledges support through the project ENGAGE funded in the framework of the Leibniz Competition (SAW-2016-PIK-1), as well as through the project CHIPS, part of AXIS, an ERA-NET initiated by JPI Climate, and funded by FORMAS (SE), DLR/BMBF (DE, grant no. 01LS19XXY), AEI (ES) and ANR (FR) with cofunding by the European Union (grant no. 776608). R.S. acknowledges the financial support from the National Council for Scientific and Technological Development (CNPq), from the National Institute of Science and Technology for Climate Change Phase 2 under CNPq grant no. 465501/2014-1 and the National Coordination for High Level Education and Training (CAPES) grant no. 88887.136402/2017-00, all from Brazil. A.M. acknowledges support from the US Department of Energy, Office of Science’s Integrated Multisector Multiscale Modelling project and National Science Foundation’s Water Sustainability and Climate grant no. 1360445. This work was authored in part by the National Renewable Energy Laboratory (A.M.), operated by Alliance for Sustainable Energy, LLC, for the US Department of Energy (DOE) under Contract No. DE-AC36-08GO28308. S.F. is supported by the Environment Research and Technology Development Fund (2-1908 and 2-2002) provided by the Environmental Restoration and Conservation Agency, Japan. C.P. is supported by Korea Environment Industry & Technology Institute (KEITI) through Climate Change R&D Programme, funded by the Korea Ministry of Environment (MOE) (2018001310003).
Author information
Authors and affiliations.
Copernicus Institute of Sustainable Development, Utrecht University, Utrecht, the Netherlands
Seleshi G. Yalew, David E. H. J. Gernaat, Ioanna Mouratiadou & Detlef P. van Vuuren
Water Systems and Global Change Group, Wageningen University, Wageningen, the Netherlands
Seleshi G. Yalew, Michelle T. H. van Vliet & Fulco Ludwig
Policy Analysis, Department of Multi-Actor Systems, Technical University of Delft, Delft, the Netherlands
Seleshi G. Yalew
Department of Physical Geography, Utrecht University, Utrecht, the Netherlands
Michelle T. H. van Vliet
Netherlands Environmental Assessment Agency-PBL, The Hague, the Netherlands
David E. H. J. Gernaat & Detlef P. van Vuuren
Advanced Science Research Center, GC/CUNY, New York City, NY, USA
Ariel Miara
National Renewable Energy Laboratory, Golden, CO, USA
Department of Landscape Architecture, College of Urban Science, University of Seoul, Seoul, Korea
International Institute for Applied Systems Analysis-IIASA, Laxenburg, Austria
Edward Byers
Fondazione CMCC, Venice, Italy
Enrica De Cian & Shouro Dasgupta
Università Ca’ Foscari Venezia, Venice, Italy
Potsdam Institute for Climate Impact Research, Leibniz Association, Potsdam, Germany
Franziska Piontek & Robert Pietzcker
Joint Global Change Research Institute, Pacific Northwest National Laboratory, College Park, MD, USA
Gokul Iyer, Mohamad Hejazi & Silvia R. Santos da Silva
MaREI Centre, Environmental Research Institute, University College Cork, Cork, Ireland
James Glynn
Institute for Sustainable Resources, University College London, London, UK
Olivier Dessens
Programa de Planejamento Energético, COPPE, Universidade Federal do Rio de Janeiro, Rio de Janeiro, Brazil
Pedro Rochedo & Roberto Schaeffer
Center for Social and Environmental Systems Research, National Institute for Environmental Studies, Tsukuba, Japan
Shinichiro Fujimori
Department of Environmental Engineering, Kyoto University, Kyoto, Japan
Laboratoire d’économie appliquée de Grenoble, Grenoble, France
Silvana Mima
Department of Atmospheric and Oceanic Science, University of Maryland, College Park, MD, USA
Silvia R. Santos da Silva
Council on Energy, Environment and Water, New Delhi, India
Vaibhav Chaturvedi
Laboratoire des Sciences du Climat et l’Environnement-LSCE, Paris, France
Robert Vautard
You can also search for this author in PubMed Google Scholar
Contributions
S.G.Y. and D.P.v.V. codesigned the study. S.G.Y. collected and analysed data, and cowrote the initial draft manuscript with D.P.v.V. S.G.Y., D.P.v.V. and M.T.H.v.V. performed sectoral analysis of energy systems. S.G.Y., D.P.v.V., M.T.H.v.V., D.E.H.J.G., F.L., A.M., C.P., E.B., E.d.C., F.P., G.I., I.M., J.G., M.H., O.D., P.R., R.P., R.S., S.F., S.D., S.M., S.R.S.d.S., V.C. and R.V. contributed to the review of sectoral and regional climate impacts.
Corresponding author
Correspondence to Seleshi G. Yalew .
Ethics declarations
Competing interests.
The authors declare no competing interests.
Additional information
Publisher’s note Springer Nature remains neutral with regard to jurisdictional claims in published maps and institutional affiliations.
Supplementary information
Supplementary information.
Supplementary Tables 1–3, Note 1 and Fig. 1.
Source data
Source data fig. 2.
Data points of articles, their category and years published.
Source Data Fig. 3
Data points of article category, warming level and scenario years.
Source Data Fig. 4
Data points of percentage changes of climate impact per region.
Rights and permissions
Reprints and permissions
About this article
Cite this article.
Yalew, S.G., van Vliet, M.T.H., Gernaat, D.E.H.J. et al. Impacts of climate change on energy systems in global and regional scenarios. Nat Energy 5 , 794–802 (2020). https://doi.org/10.1038/s41560-020-0664-z
Download citation
Received : 13 August 2019
Accepted : 01 July 2020
Published : 03 August 2020
Issue Date : October 2020
DOI : https://doi.org/10.1038/s41560-020-0664-z
Share this article
Anyone you share the following link with will be able to read this content:
Sorry, a shareable link is not currently available for this article.
Provided by the Springer Nature SharedIt content-sharing initiative
This article is cited by
Extreme weather events on energy systems: a comprehensive review on impacts, mitigation, and adaptation measures.
- Ana C. R. Gonçalves
- Xurxo Costoya
- Margarida L. R. Liberato
Sustainable Energy Research (2024)
High-resolution meteorology with climate change impacts from global climate model data using generative machine learning
- Grant Buster
- Brandon N. Benton
- Ryan N. King
Nature Energy (2024)
Sustainable pathways towards universal renewable electricity access in Africa
- Rebecca Peters
- Jürgen Berlekamp
- Christiane Zarfl
Nature Reviews Earth & Environment (2024)
Climate change impacts on the extreme power shortage events of wind-solar supply systems worldwide during 1980–2022
- Dongsheng Zheng
- Qiang Zhang
Nature Communications (2024)
Targeting net-zero emissions while advancing other sustainable development goals in China
- Wenying Chen
- Keywan Riahi
Nature Sustainability (2024)
Quick links
- Explore articles by subject
- Guide to authors
- Editorial policies
Sign up for the Nature Briefing newsletter — what matters in science, free to your inbox daily.

- Open access
- Published: 23 February 2021
A critical review of the integration of renewable energy sources with various technologies
- Erdiwansyah ORCID: orcid.org/0000-0001-8887-8755 1 , 2 ,
- Mahidin 3 ,
- H. Husin 3 ,
- Nasaruddin 4 ,
- M. Zaki 3 &
- Muhibbuddin 5
Protection and Control of Modern Power Systems volume 6 , Article number: 3 ( 2021 ) Cite this article
26k Accesses
197 Citations
1 Altmetric
Metrics details
Wind power, solar power and water power are technologies that can be used as the main sources of renewable energy so that the target of decarbonisation in the energy sector can be achieved. However, when compared with conventional power plants, they have a significant difference. The share of renewable energy has made a difference and posed various challenges, especially in the power generation system. The reliability of the power system can achieve the decarbonization target but this objective often collides with several challenges and failures, such that they make achievement of the target very vulnerable, Even so, the challenges and technological solutions are still very rarely discussed in the literature. This study carried out specific investigations on various technological solutions and challenges, especially in the power system domain. The results of the review of the solution matrix and the interrelated technological challenges are the most important parts to be developed in the future. Developing a matrix with various renewable technology solutions can help solve RE challenges. The potential of the developed technological solutions is expected to be able to help and prioritize them especially cost-effective energy. In addition, technology solutions that are identified in groups can help reduce certain challenges. The categories developed in this study are used to assist in determining the specific needs and increasing transparency of the renewable energy integration process in the future.
1 Introduction
Decentralization in the electricity sector is a major step in the spread of renewable energy sources that can reduce dependence on fossil fuels [ 56 ]. Global growth of photovoltaics (PV) and wind power in recent years has been 4% and 7%, respectively. The average increase over the past 5 years reached 27% PV and 13% wind [ 37 , 80 , 109 , 116 ]. Variable renewable energy (VRE) has differences, in various ways, from conventional generation. There are six main characteristics of VRE generator output, such as: the main resource has variable, small and modular VRE generators, which are different from conventional generators and are non-synchronous and an unpredictable type of VRE, although there may be low costs in the short-term [ 5 , 50 , 59 ]. These characteristics can create various challenges to the existing power system. In this case, power system performance characteristics can be affected because of some predefined challenges, e.g. the capacity for transmission line loss or inadequate generation. In addition, the inability of portfolio generation available for matching the demand for power to the needs at any time [ 11 , 31 , 39 , 40 , 63 , 88 , 113 , 129 ].
Existing energy technologies can be used to overcome these challenges. In this case, modification technology and renewable technology can reduce some of the effects, such as the expansion of transmission networks and centralized or distributed storage devices. Integration of VREs connected to power systems requires technological solutions to achieve the decarbonization target. However, the application of a technology can cause complications caused by three main factors. First, technology choices include the implicit or explicit application of the costs, and the maturity and technological preferences of policymakers as well as companies [ 46 , 90 , 95 , 115 ]. Second, the decision on a specific solution technology is not via a single entity but rather several actors, such as utilities, system operators and regulators [ 57 , 66 , 94 , 124 ]. Finally, designated technologies vary by region including the VRE share of generator portfolios or individual power configurations for interconnected island systems [ 21 , 69 , 82 ].
From the opinions of several practitioners and researchers on energy transition, we can say that there is not enough transparency on the scope of the technologies to overcome these challenges [ 53 , 60 , 75 ]. The individual analysis offered by some proposes specific technologies, e.g. voltage management solutions for networks distributed through VRE penetration [ 70 , 77 , 98 , 131 ]. However, there are several technologies presented in this paper that have the potential to overcome broader challenges such as battery storage. In addition, scenarios for investigating the deployment of specific technologies to increase storage and transmission capacity have also been discussed [ 33 , 49 , 101 ]. However, from several studies, the substitution effects of different technology solutions are very rarely considered. Other studies focus only on some aggregate challenges, especially the challenges of flexibility [ 10 , 74 , 81 , 84 , 110 , 118 ]. However, challenges are defined at an aggregate level such that they do not necessarily lead to a particular solution technology. While some technology solutions and individual challenges might be known, some of the available literature does not provide a transparent picture. It is very important that decision-makers and researchers alike are aware of these factors when considering energy transition. When so informed, they will be better able to determine the road map and strategy on technology for the development of power system plants.
Renewable energy technology is widely covered in the literature and clearly various challenges still exist. The review carried out in this study aims to map the challenges of VRE by describing what technology solutions are appropriate to overcome these challenges. The approach taken in this paper is the analysis of data from the literature used to compile and map the list of technology solutions and challenges based on their interrelations, and to identify any lack of consistency and classify challenges to VRE. This approach aims to distinguish the observed symptoms, e.g. performance characteristics that change. Furthermore, this analysis is complemented with information from several experts to strengthen and ensure more accurate results. The findings on challenges and their linkages to technology solutions are also discussed. The relevant implications for policymakers and companies are presented in the next section. The main contribution of this review is to provide up-to-date information and useful knowledge in the deployment of RET so that energy access across the country can be improved. The systemic approach within an RE framework for information on important components of the RE ecosystem is a feature of this article.
The outline of this paper is as follows. Part one is an overview. Part two describes the materials and methods used. Part three gives the results and discusses the review and analysis regarding RET. Part three presents the findings and solutions of RET in detail. The final part is the conclusion.
2 Materials and methodology
2.1 collecting challenges and technology solutions.
Analysis of the challenges and technological solutions contained in this study were collected from literature published in journals, conferences and from some institutions in the English language. The samples analysed in this paper were mostly collected from internationally recognized journals and sources from established publishers such as Elsevier (Science Direct), Springer, Wiley, etc. [ 13 , 38 , 117 ] and from various online websites published by several official government and private institutions and research institutions. The journals analysed and reviewed in this paper contained 132 articles deemed relevant to technological challenges and solutions, especially for renewable energy.
The literature review conducted in this paper is divided into several categories to map various technological challenges and solutions comprehensively. The first category reviewed related to challenges and technological solutions from a systemic viewpoint, looking at the differences between systematic studies that focus specifically on technological solutions and challenges as well as other foci relating to VRE in an integrated manner in certain areas such as islands or villages. Reviews relating to market share issues or regulations are set in perspective from a technological or operational solution integrated directly with VRE. The final category analysed is the basis for extracting technological solutions and challenges. Studies relating to perspective technology and operations are used to eliminate ambiguity for the identification of challenges. This is due to dependence on fundamental technical phenomena. Various sequential effects in increasing the yield of VRE penetration have been reported in several studies [ 35 , 71 , 97 , 120 ]. This is done because it does not have the marginal cost that is important to the challenges of integrating renewable energy. However, the ambiguity of challenge that is defined on the economic perspective has a lower spot price so that it is following the wishes of the community in perspective. To define various technical challenges including generation, it is inadequate to adjust ambiguity because it has potential effects that are not desired by stakeholders. For example, the selection of problems, in particular, is not an institutional or organizational challenge. As such, it is very easy to overlook storage from a technical point of view. Organizations or institutions that have changed are in fact steps for technical reconfiguration. In addition, it can increase more than one market share for technology solutions to power systems.
Integration of challenges and technological solutions collected and analysed from a variety of literature is a function as well as interview input for further research processes. This challenge is not tangible, in this case, the description and the words conveyed have differences. First, the challenges are collected in a long form, then iteratively collected and repeated. The technological solutions collected are determined with two requirements, first; independently this technology must be able to mitigate one another and automatically the challenges are integrated directly into VRE. Such requirements are very necessary to prevent the grouping of sub-technologies used as technological solutions. One example of sub-technology is Smart Meter, which is very possible to respond to requests as needed. However, it cannot independently reduce challenges that are integrated directly with VRE. Therefore, it is important to classify responses to requests for technological solutions, however, not for Smart Meters. As for the second category, it is done to define technology solutions based on their respective functions as explained by [ 16 , 76 , 93 ]. Thus, the exclusion of technological solutions can gradually be helped by the differences between one another. Given the example of the request-response, the main function of this technology is to reduce power at certain times and devices. However, response requests are operated on different devices, for example, electric heaters and heat pumps so that different technological solutions cannot serve similar functions. This study develops the challenges and technological solutions based on the various literature reviewed. The identification of all interrelated technological solutions is described with specific challenges.
The list of challenges as explained earlier will be refined with literature and reviews relating to challenges according to their level and challenges related to overall causality (Table 1 ). The relationship between the challenges and the technological solutions analysed shows that the two are mutually exclusive. Therefore, the analysis methodology applied in this study aims to find out the causes, management tools and the standard tools. Besides, the purpose of applying this method is to identify the main causes of certain problems and events as the root causes [ 14 , 36 , 112 ]. Categories with failure modes on micro-networks that can be used to find various errors and resolutions are found in the method [ 34 , 48 , 52 ]. The method is applied to identify the increasing symptoms of penetration of VRE collected from various literature. The symptoms analysed represent various effects that have adverse effects on performance characteristics for the power system. The identification of challenges found in the literature is then mapped based on the symptoms of each specific VRE characteristic that is the root of the problem.
3 Result and discussion
3.1 defiance.
There are eight categories of problems in increasing VRE penetration found in some of the literature as shown in Table 2 . Furthermore, the problems that have been identified were divided into four main categories as requirements for basic performance for power systems. The dominant performance requirement for end consumers is one of sufficient power quality. This power quality consists of a continuous and uninterruptible power supply with a steady-state of voltage and current. In addition, if there is an instant matching, it is better to stay awake and safe. The basic category of VRE can be responsible for power quality challenges that include the modularity of the VRE generator and the fact of dissonance. Furthermore, the flow was categorized as transmission and distributed power efficiency. Multiple stream categories were the cause of the challenge compared to the other categories. Modularity, location constraints and VRE were the biggest part of the flow of challenges. The frequency of controls and challenges was categorized as stability to the power system to restore the system after a blackout. The cause of the stability of this challenge was due to the modularity of the VRE generator and the synchronization of the generator. The relationship between the challenges with the balance of supply and demand for active power in the short and long term of the system was categorized into power balance. This included a wider coordination system of speed capacity in the power system to the generator and ramp to a minimum. The main cause of the challenges was the uncertainty and variability of VRE. The main problem from the results of the analysis has given a bottom-up challenge category that was consistent by adjusting the problems contained in the power system to increase VRE penetration. A detailed review of the interrelated challenges between VRE characteristics and challenges is the basis of the review in this paper.
The results of the analysis of the main problems contained in an electricity network problem that includes a mismatch of demand and electricity supply are shown in Fig. 1 . Schematic description of the analysed problem was categorized into five chains, i.e. the causal effects of different VRE characteristics. Further analysis was carried out to ascertain the level of detail of each so that the problem can be resolved as quickly as possible before the selection of challenges interrelation analysis. Demand and supply that do not have in common certainly have a variety of different reasons besides increasing VRE penetration. For example, delivery limitation from nuclear power plants and coal is one of the reasons because the power system is less flexible [ 74 ]. However, the main focus of this paper discusses the challenges and integrated technological solutions and causes of the connection to the increased VRE penetration. The main problems analysed are eight causes caused by the increased VRE penetration as summarized in Fig. 1 . A list of the challenges that has been summarized includes descriptions and categories of each as well as the symptoms observed and references as shown in Table 3 . Twenty six challenges have been identified as a whole and most of them are challenges related to power system stability and power flow.
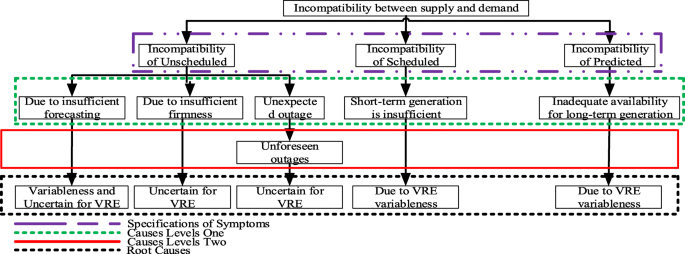
Analysing the root cause to balance challenges
3.2 Technologies of Solutions
Categorical and technological solutions and challenges are generally not specifically available in the literature. This is because most categories are implicit and have differences in the focus of each research. The study of power systems are flexible such as technology that can consume and produce power actively [ 25 , 97 ]. Meanwhile, research on electricity networks tends to focus on technology for power distribution and transmission only ([ 99 , 100 ]. Technology solutions that are comprehensively registered are not included in the technology identification as reported in the study [ 63 ]. Categorization of technology solutions is determined such as transformation in the energy sector and conclusions with a higher level. Research on top-line classification using two characteristics assigned to technological solutions has been reported by [ 54 ]. Transformations in the energy sector that lead to distributed or centralized systems are characteristics as reflected in the literature [ 19 , 22 , 26 ]. Therefore, the difference between distributed and centralized technology solutions can be used at a higher or lower level of system challenge. Technology with one side of generation and transmitted technology that is distributed with the other side can be categorized into the second as reported in several kinds of literature. Technology flexibility can be classified as technological solutions such as technology that contributes to system flexibility producing or consuming active power or better known as grid technology that is also classified as a technological solution. The characteristics of technological solutions can be divided into four groups through two assignments. The group which is categorized as two assignments includes a description, e.g. potential applications and solutions for each technology solution as shown in Table 4 . Twenty one technology solutions have been identified; 10 of which are distributed technology solutions, while the remaining 11 technological solutions are centralized. Besides, 21 technological solutions are also distinguished from the flexibility and grid technology systems. Whereas, there are 8 flexibility technologies and 13 grid technologies.
Grid technology is considered more attractive than flexibility technology because grid technology can serve both centralized and distributed systems. An estimation solution in a grid distribution system can estimate or measure a particular grid area. While responding to requests to serve multiple applications can be done with technology flexibility. Centrally distributed and distributed technology systems are very similar when they were first seen. However, more closely, the design between the two shows the difference. Where the ability to serve the application is distinguished from the operator and the owner himself. This difference is illustrated in the case of a stored and distributed system. On the other hand, storage with a distributed system is generally a battery unit installed at the household level with a closed state. Optimized independent consumption of these units is generally found in households, e.g. end consumers or stand-alone. While centralized storage systems such as water pump storage units or batteries are connected. The purpose of this application is for a short period during peak periods or to maintain the system’s power stability. Whereas centralized distributed storage is generally found in the operator or utility system.
3.3 Interrelationships between solutions to challenges
After completing the identification of technological solutions and challenges for integrated VRE, an analysis was carried to overcome the challenges as shown in Table 5 . Challenges contained in the scope of solutions can ignore the number of technological solutions so that defined challenges can be addressed. Successful solution spaces are identified as illustrated in Table 6 . Where the potential solutions contained in technological solutions that refer to several challenges can be addressed as quickly as possible. Because the space and potential of qualitative solutions are numerical comparisons and very limited to be used. Observation matrices made from the perspective of solutions such as high potential solutions and overall challenges are technological flexibility. VRE generators and distributed conventional generators that have a high level of potential solutions are included in the flexibility technology group, for example, large conventional generators with low potential solutions and conventional generation. Furthermore, distributed technological solutions tend to be higher compared to centralized systems. However, distributed grid technology has special exceptions especially for limiter or harmonic filter devices. Finally, the unique value that grid technology has on specific challenges include direct current control systems that have high voltage (HVDC) and power flow that can accurately solve problems such as long transmission distances. However, these challenges can generally be addressed by utilizing flexible technology.
Contributions made by the solution technology to solve the challenges are described in Tables 5 and 6 . Challenges that are local and site-specific have a narrower scope because the solution can only be done by the distributed solution technology, modified distributed VRE generators or additional technology solutions, e.g. harmonic filter. The whole technology group can solve various flow challenges, except technology-centred flexibility that has limitations in solving flow problems. The difference in solution space is included in the category of flow challenges starting from a narrow space to a wider space. The challenge of stability can be solved by a system technology solution by controlling at the system level centrally. Thus, the challenges of flow and distributed technology networks cannot solve challenges to stability, unless the system level can be aggregated. Stability categories such as challenges have wider solution space; however, systems in control interactions cannot be improved. To be able to balance, challenges can only be done by flexibility technology so that existing challenges can be tailored to the needs and active power consumption, excerpt for the increase in the more important VRE estimates. In general, the challenges in the balance category have a wider solution space than the availability of generations in the long run.
Three insights are very important in integrating VRE and decarbonization for the energy sector. The first process discusses two insights for overcoming integrated VRE challenges, e.g. a different power system. The last insight illustrates the results of research that can improve policymaking in the energy sector transition. Solution space for different challenges is the first point, while earlier observations are made for several types of technology that can solve specific challenges. However, the intuitive analysis of the results of expert interviews shows that business people and policymakers are not very familiar with the technological solutions that can be used to solve certain challenges. It is very clear that this technology falls into different categories. However, the development of different solution technologies can reduce the economic viability of a single technology and diminish market potential. Contributions in the decline in market price levels have a relationship with the things mentioned above. This is the same as the balancing power market in Germany. In this case, storage institutional frameworks, increasing VRE forecasts, changing demand responses simultaneously can significantly reduce market prices [ 43 , 51 , 87 ].
An illustration of the balance and challenges of stability can be used further as an example. The results of the interviews with experts clearly show that each different technology category can function as technology e.g. request responses available only focus on a centralized solution. Therefore, large scale and conventional generation are competitive technologies. However, the distribution of technological flexibility is not focused on analysing the more competitive technological landscape. This can be said as a prominent relationship to the potential influence of grid technology on technology flexibility, e.g. VRE estimates that increase significantly. This is because the size of the market is reduced to the demand response and storage technology. Technology like this, in general, can be used as a counterweight to a certain size of the market by looking at the quality of market participants. Lack of knowledge of technology and its groups is the main reason since competitive technology can be used for decision-making information for processes in a smoother energy transition.
The distribution of solution technology portfolios in each region for VRE integration contained in the literature seems to be very generic. Thus, the guidance given to companies and policymakers always fails to develop business policies and strategies. For future decision making, it can be assisted through an interrelation matrix such as preparing proposals and technology roadmaps both nationally and internationally. This aims to be able to decarbonize the energy sector. Interrelation material functions to match each category as well as some of the history of each country. Every quality challenge has occurred regionally for high distributed VRE penetration so that the spread of flexibility is needed especially distributed technology networks. Countries with a high penetration of VRE generators are southern England, southern and northern Italy and southern Germany [ 109 ]. Although the availability of data spread flexibility is not available for distributed technology networks in certain regions, projects such as the RD&D smart grid are technologies with very high priority for policymakers and companies in these countries [ 24 , 28 , 78 ]. The challenge of flow for the transmission rate reached by countries such as Germany, in general, requires a technology system with a centralized network. Such systems, such as transmission networks or amplifications, must be expanded, active power control and HVDC transmission systems. Germany is currently preparing several large projects that can be utilized by using technology. This is done after the assessment phase in determining the design and size of the complex installation has been completed.
Countries such as Ireland and Spain have done similar things, both of which have faced stability challenges. On the other hand, the transmission operator system is set as the centralized controller of the VRE generator. It aims to the needs of VRE generators to support network stability [ 3 , 102 , 108 ]. Besides, the investigation was carried out to ease the limitation of the stability criteria. Finally, solving the challenge of balance can only be done through technology flexibility. California, for example, is a country that have difficulty of being able to maintain power balance when the sun changes night so that the VRE generation has decreased significantly [ 32 ]. To encourage investment in storage with more flexible generators and environmentally friendly renewable energy, the State of California has introduced several new products [ 2 , 29 , 30 ]. Thus, interrelation matrix can be concluded that its function can be carried out by business people and those who make policies in identifying solutions technology groups. Finally, the challenges that are prevalent in certain areas can be reduced and the formulation of steps and policy strategies in supporting the dissemination of technology can be easily carried out.
Frequent debates between actors to prioritize technological solutions in VRE and irrigation management in the energy sector have often been carried out. Priority for technology solutions in integrating VRE with costs and ease of implementation is reported by several researchers ([ 21 , 35 , 99 , 100 ]. This perspective has short-term benefits, also, the potential solutions that are perpetuated from this perspective are differences in facing challenges. Technology solutions are prioritized based on their respective solutions so that technology flexibility can be used as a solution to the challenges of VRE. This is as stated by experts in supporting the potential of technological flexibility ([ 99 , 100 , 126 ]). The results of the analysis can support the call for decision-makers adjusted to market rules or the placement of newly applied policies. Remuneration schemes for reactive power are introduced in the regional market. However, technology ratings are determined solely based on their respective potential and do not take into account other technological solutions that contribute to solving challenges. Besides, the solution space is different among all challenges. To consider these factors, the ranking of technologies can be adjusted to their potential in solving challenges. The preference for the deployment of this flexibility technology is specifically found in distributed and centralized VRE. Protection strategies with appropriate equipment can solve specific challenges, and higher interests can be achieved by the following perspectives. Response to requests both small and large is part of the technology solution. In addition, there are large generators with lower priority because of the limitations of the potential for more unique solutions. Relevantly to distinguish VRE integration, there are two examples large, small demand response spreads and large flexible conventional generators. Cost savings from existing solutions can be realized in the short term. However, it is not enough to only deal with the scope of the existing challenges or potential. The aspects discussed can be assumed to confirm the benefits of the results of the analysis for policymakers as a whole.
The results of the analysis carried out have important limitations to be considered when interpreting the final results. A review of specific research on existing challenges can improve VRE penetration. However, additional challenges which are not listed in this study can also face challenges such as the electric power system. At the same time, analysis of challenges was also found in power systems with lower VRE penetration. Specifically, the analysis conducted in this study is a challenge that is directly related to technology solutions. This analysis does not measure one technology solution that can solve only certain challenges. In addition, the future developments beyond the scope of this analysis can be reduced, e.g. the emergence of new solution technologies that can change frequency stability criteria or more robust end-user equipment such as variable frequency drives. Furthermore, the specific costs of the solution technology, the urgency of the challenges or the feasibility of implementing the solution technology are not considered. This is due to environmental constraints such as high land, social areas such as the public for receiving the final transmission line. This quantification is adapted to specific contexts with differences in power system characteristics. Furthermore, high levels of uncertainty are more vulnerable when considered such as revenue and costs than differences in applications and technology solutions. This need is needed for the need to think in grouping portfolios or technologies that focus on the completion of integrated VRE.
4 Conclusion
Specifically, the review in this research is to study the integration of VRE systems that are connected with modern power systems and technology to overcome challenges. Besides, the need for power system technology in increasing VRE market share with complex integration is also discussed. The collection of challenges undertaken in this study was drawn from a variety of literature relating to technology solutions in integrating VRE. The challenges developed can consistently integrate VRE which is the root problem of this analysis. The results of this analysis are supplemented by data from interviews of experts who have helped in investigations related to technology solutions and their challenges.
The results of the analysis with some insights outlined in the study can be summarized as follows
VRE integrated with challenges can affect the characteristics of the power system.
Technology solutions that vary with the number of challenges can be significantly overcome. In general, technology flexibility has a higher solution potential than the use of grid technology.
The identified technological solution facilities are intended to be able to overcome challenges in several categories.
Identification of challenges from various practice literature can be arranged and collected based on the root of the problem to produce each of the more exclusive challenge categories.
Categories and collections of technology solutions are used to test challenges that can be overcome by a single technology.
The size of potential solutions becomes very important for companies or policymakers in promoting certain technologies and their respective solutions.
Some of the descriptions presented in this review are a starting point for future research related to this topic. The relationship between technology solutions and challenges is one of the new fields of research. This is done with an estimated cost compared to the use of different solution technologies and can be introduced comparatively to the environment as a whole. Life Cycle Assessment (LCA) can be used to measure costs integrated with VRE because the installed capacity with future projections is available [ 41 , 107 , 114 , 125 ]. This system can significantly improve recommendations on policies issued. Overall, the development of individual solutions technology that is integrated with VRE is an issue that has a high price for the transition in the energy sector in a sustainable manner. In this case, a further investigation between the characteristics of different power systems and geographies is on one side of the technology solutions and challenges with different sides.
5 Nomenclature
VRE Variable Renewable energy
HVDC High-Voltage Direct Current
RE Renewable Energy
LCA Life Cycle Assessment
RET Renewable Energy Technology
PV Photovoltaics
Abdelshafy, A. M., Jurasz, J., Hassan, H., & Mohamed, A. M. (2020). Optimized energy management strategy for grid connected double storage (pumped storage-battery) system powered by renewable energy resources. Energy , 192 , 116615. https://doi.org/10.1016/j.energy.2019.116615 .
Article Google Scholar
Abdul-Rahman, K. H., Alarian, H., Rothleder, M., Ristanovic, P., Vesovic, B., & Lu, B. (2012). Enhanced system reliability using flexible ramp constraint in CAISO market. In 2012 IEEE power and energy society general meeting , (pp. 1–6). https://doi.org/10.1109/PESGM.2012.6345371 .
Chapter Google Scholar
Ackermann, T., Martensen, N., Brown, T., Schierhorn, P. P., Boshell, F., Gafaro, F., & Ayuso, M. (2016). Scaling up variable renewable power: The role of grid codes World Future Energy.
Google Scholar
Agency, I. E. (2005). Variability of wind power and other renewables: Management options and strategies International Energy Agency.
Agency, I. E. (2014). The power of transformation: Wind, sun and the economics of flexible power systems IEA.
Book Google Scholar
Alanazi, M., Mahoor, M., & Khodaei, A. (2020). Co-optimization generation and transmission planning for maximizing large-scale solar PV integration. International Journal of Electrical Power & Energy Systems , 118 , 105723. https://doi.org/10.1016/j.ijepes.2019.105723 .
Alet, P.-J., Baccaro, F., De Felice, M., Efthymiou, V., Mayr, C., Graditi, G., … Tselepis, S. (2015). Quantification, challenges and outlook of PV integration in the power system: A review by the European PV technology platform EU PVSEC 2015.
Al-Haddad, K. (2010). Power quality issues under constant penetration rate of renewable energy into the electric network. In Proceedings of 14th international power electronics and motion control conference EPE-PEMC 2010 , (pp. S11-39–S11-49). https://doi.org/10.1109/EPEPEMC.2010.5606699 .
Alizadeh, M. I., Parsa Moghaddam, M., Amjady, N., Siano, P., & Sheikh-El-Eslami, M. K. (2016). Flexibility in future power systems with high renewable penetration: A review. Renewable and Sustainable Energy Reviews , 57 , 1186–1193. https://doi.org/10.1016/j.rser.2015.12.200 .
Allard, S., Debusschere, V., Mima, S., Quoc, T. T., Hadjsaid, N., & Criqui, P. (2020). Considering distribution grids and local flexibilities in the prospective development of the European power system by 2050. Applied Energy , 270 , 114958. https://doi.org/10.1016/j.apenergy.2020.114958 .
Al-Shetwi, A. Q., Hannan, M. A., Jern, K. P., Mansur, M., & Mahlia, T. M. I. (2020). Grid-connected renewable energy sources: Review of the recent integration requirements and control methods. Journal of Cleaner Production , 253 , 119831. https://doi.org/10.1016/j.jclepro.2019.119831 .
Al-Shetwi, A. Q., Sujod, M. Z., Blaabjerg, F., & Yang, Y. (2019). Fault ride-through control of grid-connected photovoltaic power plants: A review. Solar Energy , 180 , 340–350. https://doi.org/10.1016/j.solener.2019.01.032 .
Analytics, C. (2020). Web of Science. Retrieved from https://login.webofknowledge.com/error/Error?Src=IP&Alias=WOK5&Error=IPError&Params=%26Error%3DClient.NullSessionID&PathInfo=%2F&RouterURL , https://%3A%2F%2Fwww.webofknowledge.com%2F&Domain=.webofknowledge.com
Andersen, B., & Fagerhaug, T. (2006). Root cause analysis: Simplified tools and techniques . Quality Press; Journal for Healthcare Quality. https://journals.lww.com/jhqonline/Citation/2002/05000/Root_Cause_Analysis__Simplified_Tools_and.12.aspx .
Armghan, H., Yang, M., Wang, M. Q., Ali, N., & Armghan, A. (2020). Nonlinear integral backstepping based control of a DC microgrid with renewable generation and energy storage systems. International Journal of Electrical Power & Energy Systems , 117 , 105613. https://doi.org/10.1016/j.ijepes.2019.105613 .
Arthur, W. B. (2009). The nature of technology: What it is and how it evolves . Simon and Schuster. https://www.books.google.co.id/books?hl=en&lr=&id=3qHs-XYXN0EC&oi=fnd&pg=PA1&dq=The+nature+of+technology:+What+it+is+and+how+it+evolves&ots=5ZNboK7VAf&sig=KJ8N_DMgENEfOAU-wGRAlXUjMEw&redir_esc=y#v=onepage&q=The%20nature%20of%20technology%3A%20What%20andit%20is%20how%20andit%20is%2020evolves&f=false .
Bartolini, A., Carducci, F., Munoz, C. B., & Comodi, G. (2020). Energy storage and multi energy systems in renewable energy communities with high renewable energy penetration. Renewable Energy . https://doi.org/10.1016/j.renene.2020.05.131 .
Batalla-Bejerano, J., & Trujillo-Baute, E. (2016). Impacts of intermittent renewable generation on electricity system costs. Energy Policy , 94 , 411–420. https://doi.org/10.1016/j.enpol.2015.10.024 .
Battaglini, A., Lilliestam, J., Haas, A., & Patt, A. (2009). Development of SuperSmart grids for a more efficient utilisation of electricity from renewable sources. Journal of Cleaner Production , 17 (10), 911–918. https://doi.org/10.1016/j.jclepro.2009.02.006 .
Bazilian, M., Denny, E., & O’Malley, M. (2004). Challenges of increased wind energy penetration in Ireland. Wind Engineering , 28 (1), 43–55.
Bird, L., Milligan, M., & Lew, D. (2013). Integrating variable renewable energy: Challenges and solutions . Golden: National Renewable Energy lab.(NREL).
Blarke, M. B., & Jenkins, B. M. (2013). SuperGrid or SmartGrid: Competing strategies for large-scale integration of intermittent renewables? Energy Policy , 58 , 381–390. https://doi.org/10.1016/j.enpol.2013.03.039 .
Cailliau, M., Ogando, J. A., Egeland, H., Ferreira, R., Feuk, H., Figel, F., … Villar, C. M. (2010). Integrating intermittent renewable sources into the eu electricity system by 2020: Challenges and solutions . Brussels: Union of the Electricity Industry [EURELECTRIC].
Cambini, C., Meletiou, A., Bompard, E., & Masera, M. (2016). Market and regulatory factors influencing smart-grid investment in Europe: Evidence from pilot projects and implications for reform. Utilities Policy , 40 , 36–47. https://doi.org/10.1016/j.jup.2016.03.003 .
Chandler, H. (2011). Harnessing variable renewables: A guide to the balancing challenge . Paris: International Energy Agency.
Child, M., Kemfert, C., Bogdanov, D., & Breyer, C. (2019). Flexible electricity generation, grid exchange and storage for the transition to a 100% renewable energy system in Europe. Renewable Energy , 139 , 80–101. https://doi.org/10.1016/j.renene.2019.02.077 .
Cifor, A., Denholm, P., Ela, E., Hodge, B.-M., & Reed, A. (2015). The policy and institutional challenges of grid integration of renewable energy in the western United States. Utilities Policy , 33 , 34–41. https://doi.org/10.1016/j.jup.2014.11.001 .
Colak, I., Fulli, G., Sagiroglu, S., Yesilbudak, M., & Covrig, C.-F. (2015). Smart grid projects in Europe: Current status, maturity and future scenarios. Applied Energy , 152 , 58–70. https://doi.org/10.1016/j.apenergy.2015.04.098 .
Cornelius, A., Bandyopadhyay, R., & Patiño-Echeverri, D. (2018). Assessing environmental, economic, and reliability impacts of flexible ramp products in MISO’s electricity market. Renewable and Sustainable Energy Reviews , 81 , 2291–2298. https://doi.org/10.1016/j.rser.2017.06.037 .
Cui, M., & Zhang, J. (2018). Estimating ramping requirements with solar-friendly flexible ramping product in multi-timescale power system operations. Applied Energy , 225 , 27–41. https://doi.org/10.1016/j.apenergy.2018.05.031 .
Das, P., Mathuria, P., Bhakar, R., Mathur, J., Kanudia, A., & Singh, A. (2020). Flexibility requirement for large-scale renewable energy integration in Indian power system: Technology, policy and modeling options. Energy Strategy Reviews , 29 , 100482. https://doi.org/10.1016/j.esr.2020.100482 .
Denholm, P., O’Connell, M., Brinkman, G., & Jorgenson, J. (2015). Overgeneration from solar energy in California. A field guide to the duck chart . Golden: National Renewable Energy lab.(NREL).
Díaz, G., Coto, J., & Gómez-Aleixandre, J. (2019). Optimal operation value of combined wind power and energy storage in multi-stage electricity markets. Applied Energy , 235 , 1153–1168. https://doi.org/10.1016/j.apenergy.2018.11.035 .
Dileep, G. (2020). A survey on smart grid technologies and applications. Renewable Energy , 146 , 2589–2625. https://doi.org/10.1016/j.renene.2019.08.092 .
DNV, G. L (2014). Integration of renewable energy in Europe, Bonn .
Douglas-Smith, D., Iwanaga, T., Croke, B. F. W., & Jakeman, A. J. (2020). Certain trends in uncertainty and sensitivity analysis: An overview of software tools and techniques. Environmental Modelling and Software , 124 , 104588. https://doi.org/10.1016/j.envsoft.2019.104588 .
EIA (2020). Installed electricity capacity Retrieved from https://www.eia.gov/international/data/world .
Elsevier (2020). ScienceDirect Retrieved from https://www.sciencedirect.com/ .
Erdiwansyah, M., Mamat, R., Sani, M. S. M., Khoerunnisa, F., & Kadarohman, A. (2019). Target and demand for renewable energy across 10 ASEAN countries by 2040. The Electricity Journal , 32 (10), 106670. https://doi.org/10.1016/J.TEJ.2019.106670 .
Erdiwansyah, Mamat, R., Sani, M. S. M., & Sudhakar, K. (2019). Renewable energy in Southeast Asia: Policies and recommendations. Science Total Environment . https://doi.org/10.1016/j.scitotenv.2019.03.273 .
Finnveden, G., Hauschild, M. Z., Ekvall, T., Guinée, J., Heijungs, R., Hellweg, S., … Suh, S. (2009). Recent developments in life cycle assessment. Journal of Environmental Management , 91 (1), 1–21. https://doi.org/10.1016/j.jenvman.2009.06.018 .
Forbes, K. F., & Zampelli, E. M. (2019). Wind energy, the price of carbon allowances, and CO2 emissions: Evidence from Ireland. Energy Policy , 133 , 110871. https://doi.org/10.1016/j.enpol.2019.07.007 .
Gan, L., Jiang, P., Lev, B., & Zhou, X. (2020). Balancing of supply and demand of renewable energy power system: A review and bibliometric analysis. Sustainable Futures , 2 , 100013. https://doi.org/10.1016/j.sftr.2020.100013 .
Ghenai, C., & Bettayeb, M. (2019). Grid-tied solar PV/fuel cell hybrid power system for university building. Energy Procedia , 159 , 96–103. https://doi.org/10.1016/j.egypro.2018.12.025 .
González, A., Daly, G., & Gleeson, J. (2016). Congested spaces, contested scales – A review of spatial planning for wind energy in Ireland. Landscape and Urban Planning , 145 , 12–20. https://doi.org/10.1016/j.landurbplan.2015.10.002 .
Hadjilambrinos, C. (2000). Understanding technology choice in electricity industries: A comparative study of France and Denmark. Energy Policy , 28 (15), 1111–1126. https://doi.org/10.1016/S0301-4215(00)00067-7 .
Hannan, M. A., Tan, S. Y., Al-Shetwi, A. Q., Jern, K. P., & Begum, R. A. (2020). Optimized controller for renewable energy sources integration into microgrid: Functions, constraints and suggestions. Journal of Cleaner Production , 256 , 120419. https://doi.org/10.1016/j.jclepro.2020.120419 .
Hare, J., Shi, X., Gupta, S., & Bazzi, A. (2016). Fault diagnostics in smart micro-grids: A survey. Renewable and Sustainable Energy Reviews , 60 , 1114–1124. https://doi.org/10.1016/j.rser.2016.01.122 .
Hedegaard, K., & Meibom, P. (2012). Wind power impacts and electricity storage – A time scale perspective. Renewable Energy , 37 (1), 318–324. https://doi.org/10.1016/j.renene.2011.06.034 .
Hirth, L., & Müller, S. (2016). System-friendly wind power: How advanced wind turbine design can increase the economic value of electricity generated through wind power. Energy Economics , 56 , 51–63. https://doi.org/10.1016/j.eneco.2016.02.016 .
Hirth, L., & Ziegenhagen, I. (2015). Balancing power and variable renewables: Three links. Renewable and Sustainable Energy Reviews , 50 , 1035–1051. https://doi.org/10.1016/j.rser.2015.04.180 .
Hlalele, T. S., Sun, Y., & Wang, Z. (2019). Faults classification and identification on smart grid: Part-a status review. Procedia Manufacturing , 35 , 601–606. https://doi.org/10.1016/j.promfg.2019.05.085 .
Holttinen, H. (2012). Wind integration: Experience, issues, and challenges. Wiley Interdisciplinary Reviews: Energy and Environment , 1 (3), 243–255.
Houseman, D. (2009). True integration challenges for distributed resources in the distribution grid. In CIRED 2009-20th international conference and exhibition on electricity distribution-part 1 , (pp. 1–4). IET. https://ieeexplore.ieee.org/abstract/document/5255832 .
Ilisiu, D., Munteanu, C., & Topa, V. (2009). Renewable integration in Romanian power system, challenge for Transelectrica company. In 2009 international conference on clean electrical power , (pp. 710–714). IEEE. https://ieeexplore.ieee.org/abstract/document/5211974 .
IPCC (2001). Climate change 2001: The scientific basis. Contribution of working group I to the third assessment report of the Interngovernmental panel on climate change .
Islam, M. R., Lu, H., Hossain, M. J., & Li, L. (2019). Mitigating unbalance using distributed network reconfiguration techniques in distributed power generation grids with services for electric vehicles: A review. Journal of Cleaner Production , 239 , 117932. https://doi.org/10.1016/j.jclepro.2019.117932 .
Jayaweera, D. (2016). Smart power systems and renewable energy system integration . Springer. https://link.springer.com/book/10.1007%2F978-3-319-30427-4 .
Javed, M. S., Ma, T., Jurasz, J., & Amin, M. Y. (2020). Solar and wind power generation systems with pumped hydro storage: Review and future perspectives. Renewable Energy , 148 , 176–192. https://doi.org/10.1016/j.renene.2019.11.157 .
Jonaitis, A., Gudzius, S., Morkvenas, A., Azubalis, M., Konstantinaviciute, I., Baranauskas, A., & Ticka, V. (2018). Challenges of integrating wind power plants into the electric power system: Lithuanian case. Renewable and Sustainable Energy Reviews , 94 , 468–475. https://doi.org/10.1016/j.rser.2018.06.032 .
Karbouj, H., Rather, Z. H., Flynn, D., & Qazi, H. W. (2019). Non-synchronous fast frequency reserves in renewable energy integrated power systems: A critical review. International Journal of Electrical Power & Energy Systems , 106 , 488–501. https://doi.org/10.1016/j.ijepes.2018.09.046 .
Karimi, M., Mokhlis, H., Naidu, K., Uddin, S., & Bakar, A. H. A. (2016). Photovoltaic penetration issues and impacts in distribution network – A review. Renewable and Sustainable Energy Reviews , 53 , 594–605. https://doi.org/10.1016/j.rser.2015.08.042 .
Kassakian, J. G., Schmalensee, R., Desgroseilliers, G., Heidel, T. D., Afridi, K., Farid, A., … Kirtley, J. (2011). The future of the electric grid , (pp. 197–234). Massachusetts Institute of Technology, Tech Rep. http://energy.mit.edu/research/future-electric-grid/ .
Katiraei, F., & Agüero, J. R. (2011). Solar PV integration challenges. IEEE Power and Energy Magazine , 9 (3), 62–71. https://doi.org/10.1109/MPE.2011.940579 .
Kayalvizhi, S., & Vinod Kumar, D. M. (2018). Optimal planning of active distribution networks with hybrid distributed energy resources using grid-based multi-objective harmony search algorithm. Applied Soft Computing , 67 , 387–398. https://doi.org/10.1016/j.asoc.2018.03.009 .
Kharrazi, A., Sreeram, V., & Mishra, Y. (2020). Assessment techniques of the impact of grid-tied rooftop photovoltaic generation on the power quality of low voltage distribution network - a review. Renewable and Sustainable Energy Reviews , 120 , 109643. https://doi.org/10.1016/j.rser.2019.109643 .
Krauter, S. (2018). Simple and effective methods to match photovoltaic power generation to the grid load profile for a PV based energy system. Solar Energy, 159, 768–776. do: https://doi.org/10.1016/j.solener.2017.11.039
Krauter, S., & Japs, E. (2014). Integration of PV into the energy system: Challenges and measures for generation and load management. In 2014 IEEE 40th photovoltaic specialist conference (PVSC) , (pp. 3123–3128). IEEE.
Kumar, A., & Pan, S.-Y. (2020). Opportunities and challenges for renewable energy integrated water-energy nexus technologies. Water-Energy Nexus . https://doi.org/10.1016/j.wen.2020.03.006 .
Lahaçani, N. A., Aouzellag, D., & Mendil, B. (2010). Contribution to the improvement of voltage profile in electrical network with wind generator using SVC device. Renewable Energy , 35 (1), 243–248. https://doi.org/10.1016/j.renene.2009.04.020 .
Li, Y., Liu, H., Fan, X., & Tian, X. (2020). Engineering practices for the integration of large-scale renewable energy VSC-HVDC systems. Global Energy Interconnection , 3 (2), 149–157. https://doi.org/10.1016/j.gloei.2020.05.007 .
Liang, X. (2017). Emerging power quality challenges due to integration of renewable energy sources. IEEE Transactions on Industry Applications , 53 (2), 855–866. https://doi.org/10.1109/TIA.2016.2626253 .
Liebensteiner, M., & Wrienz, M. (2020). Do intermittent renewables threaten the electricity supply security? Energy Economics , 87 , 104499. https://doi.org/10.1016/j.eneco.2019.104499 .
Lund, P. D., Lindgren, J., Mikkola, J., & Salpakari, J. (2015). Review of energy system flexibility measures to enable high levels of variable renewable electricity. Renewable and Sustainable Energy Reviews , 45 , 785–807. https://doi.org/10.1016/j.rser.2015.01.057 .
Luo, K., Shi, W., & Wang, W. (2020). Extreme scenario extraction of a grid with large scale wind power integration by combined entropy-weighted clustering method. Global Energy Interconnection , 3 (2), 140–148. https://doi.org/10.1016/j.gloei.2020.05.006 .
Lyons, G. (2002). Internet: Investigating new technology’s evolving role, nature and effects on transport. Transport Policy , 9 (4), 335–346. https://doi.org/10.1016/S0967-070X(02)00023-9 .
Maddaloni, J. D., Rowe, A. M., & van Kooten, G. C. (2009). Wind integration into various generation mixtures. Renewable Energy , 34 (3), 807–814. https://doi.org/10.1016/j.renene.2008.04.019 .
Malik, A. S., Albadi, M., Al-Jabri, M., Bani-Araba, A., Al-Ameri, A., & Al Shehhi, A. (2018). Smart grid scenarios and their impact on strategic plan—A case study of Omani power sector. Sustainable Cities and Society , 37 , 213–221. https://doi.org/10.1016/j.scs.2017.11.015 .
Marinescu, C., & Serban, I. (2013). About the main frequency control issues in microgrids with renewable energy sources. In 2013 international conference on clean electrical power (ICCEP) , (pp. 145–150). https://doi.org/10.1109/ICCEP.2013.6586981 .
Marques, A. C., Fuinhas, J. A., & Pereira, D. S. (2019). The dynamics of the short and long-run effects of public policies supporting renewable energy: A comparative study of installed capacity and electricity generation. Economic Analysis and Policy , 63 , 188–206. https://doi.org/10.1016/j.eap.2019.06.004 .
McPherson, M., Harvey, L. D. D., & Karney, B. (2017). System design and operation for integrating variable renewable energy resources through a comprehensive characterization framework. Renewable Energy , 113 , 1019–1032. https://doi.org/10.1016/j.renene.2017.06.071 .
McPherson, M., & Stoll, B. (2020). Demand response for variable renewable energy integration: A proposed approach and its impacts. Energy , 197 , 117205. https://doi.org/10.1016/j.energy.2020.117205 .
Mohamed, A. A. S., El-Sayed, A., Metwally, H., & Selem, S. I. (2020). Grid integration of a PV system supporting an EV charging station using Salp swarm optimization. Solar Energy , 205 , 170–182. https://doi.org/10.1016/j.solener.2020.05.013 .
Moreno-Leiva, S., Haas, J., Junne, T., Valencia, F., Godin, H., Kracht, W., … Eltrop, L. (2020). Renewable energy in copper production: A review on systems design and methodological approaches. Journal of Cleaner Production , 246 , 118978. https://doi.org/10.1016/j.jclepro.2019.118978 .
MSB, I. E. C (2012). Grid integration of large-capacity renewable energy sources and use of large-capacity electrical energy storage, white paper .
Muzhikyan, A., Muhanji, S. O., Moynihan, G. D., Thompson, D. J., Berzolla, Z. M., & Farid, A. M. (2019). The 2017 ISO New England system operational analysis and renewable energy integration study (SOARES). Energy Reports , 5 , 747–792. https://doi.org/10.1016/j.egyr.2019.06.005 .
Nadjaran Toosi, A., Qu, C., de Assunção, M. D., & Buyya, R. (2017). Renewable-aware geographical load balancing of web applications for sustainable data centers. Journal of Network and Computer Applications , 83 , 155–168. https://doi.org/10.1016/j.jnca.2017.01.036 .
Navon, A., Kulbekov, P., Dolev, S., Yehuda, G., & Levron, Y. (2020). Integration of distributed renewable energy sources in Israel: Transmission congestion challenges and policy recommendations. Energy Policy , 140 , 111412. https://doi.org/10.1016/j.enpol.2020.111412 .
Nwaigwe, K. N., Mutabilwa, P., & Dintwa, E. (2019). An overview of solar power (PV systems) integration into electricity grids. Materials Science for Energy Technologies , 2 (3), 629–633. https://doi.org/10.1016/j.mset.2019.07.002 .
Odeh, R. P., & Watts, D. (2019). Impacts of wind and solar spatial diversification on its market value: A case study of the Chilean electricity market. Renewable and Sustainable Energy Reviews , 111 , 442–461. https://doi.org/10.1016/j.rser.2019.01.015 .
O’Flaherty, M., Riordan, N., O’Neill, N., & Ahern, C. (2014). A quantitative analysis of the impact of wind energy penetration on electricity prices in Ireland. Energy Procedia , 58 , 103–110. https://doi.org/10.1016/j.egypro.2014.10.415 .
Ouai, A., Mokrani, L., Machmoum, M., & Houari, A. (2018). Control and energy management of a large scale grid-connected PV system for power quality improvement. Solar Energy , 171 , 893–906. https://doi.org/10.1016/j.solener.2018.06.106 .
Pansera, M. (2010). The nature of technology: What it is and how it evolves, William Brian Arthur, free press, Nueva York (2009), 237 pp. Investigaciones de Historia Económica , 6 (18), 200–202. https://doi.org/10.1016/S1698-6989(10)70080-1 .
Passey, R., Spooner, T., MacGill, I., Watt, M., & Syngellakis, K. (2011). The potential impacts of grid-connected distributed generation and how to address them: A review of technical and non-technical factors. Energy Policy , 39 (10), 6280–6290. https://doi.org/10.1016/j.enpol.2011.07.027 .
Pearre, N., & Swan, L. (2020). Combining wind, solar, and in-stream tidal electricity generation with energy storage using a load-perturbation control strategy. Energy , 203 , 117898. https://doi.org/10.1016/j.energy.2020.117898 .
Peterson, C. R., & Ros, A. J. (2018). The future of the electric grid and its regulation: Some considerations. The Electricity Journal , 31 (2), 18–25. https://doi.org/10.1016/j.tej.2018.02.001 .
Pierre, I., Bauer, F., Blasko, R., Dahlback, N., Dumpelmann, M., Kainurinne, K., … Romano, D. (2011). Flexible generation: Backing up renewables, Brussels .
Qiu, Y., Li, Q., Pan, Y., Yang, H., & Chen, W. (2019). A scenario generation method based on the mixture vine copula and its application in the power system with wind/hydrogen production. International Journal of Hydrogen Energy , 44 (11), 5162–5170. https://doi.org/10.1016/j.ijhydene.2018.09.179 .
Rehtanz, C., Greve, M., Häger, U., Hilbrich, D., Kippelt, S., Kubis, A., … Schwippe, J. (2014a,b). Dena ancillary services study 2030. In Security and reliability of a power supply with a high percentage of renewable energy .
Reichenberg, L., Hedenus, F., Odenberger, M., & Johnsson, F. (2018). Tailoring large-scale electricity production from variable renewable energy sources to accommodate baseload generation in europe. Renewable Energy , 129 , 334–346. https://doi.org/10.1016/j.renene.2018.05.014 .
Robles, E., Haro-Larrode, M., Santos-Mugica, M., Etxegarai, A., & Tedeschi, E. (2019). Comparative analysis of European grid codes relevant to offshore renewable energy installations. Renewable and Sustainable Energy Reviews , 102 , 171–185. https://doi.org/10.1016/j.rser.2018.12.002 .
Rothleder, M., & Loutan, C. (2017). Chapter 6 - case study–renewable integration: Flexibility requirement, potential Overgeneration, and frequency response challenges. In E. Jones (Ed.), L. e. B. t.-r. E. I , (2nd ed., pp. 69–81). Boston: Academic. https://doi.org/10.1016/B978-0-12-809592-8.00006-8 .
Ruiz-Romero, S., Colmenar-Santos, A., Mur-Pérez, F., & López-Rey, Á. (2014). Integration of distributed generation in the power distribution network: The need for smart grid control systems, communication and equipment for a smart city — Use cases. Renewable and Sustainable Energy Reviews , 38 , 223–234. https://doi.org/10.1016/j.rser.2014.05.082 .
Sajadi, A., Strezoski, L., Strezoski, V., Prica, M., & Loparo, K. A. (2019). Integration of renewable energy systems and challenges for dynamics, control, and automation of electrical power systems. Wiley Interdisciplinary Reviews: Energy and Environment , 8 (1), e321.
Sanchez-Hidalgo, M.-A., & Cano, M.-D. (2018). A survey on visual data representation for smart grids control and monitoring. Sustainable Energy, Grids and Networks , 16 , 351–369. https://doi.org/10.1016/j.segan.2018.09.007 .
Santos, R., Aguiar Costa, A., Silvestre, J. D., & Pyl, L. (2020). Development of a BIM-based environmental and economic life cycle assessment tool. Journal of Cleaner Production , 265 , 121705. https://doi.org/10.1016/j.jclepro.2020.121705 .
Sato, H., & Yan, X. L. (2019). Study of an HTGR and renewable energy hybrid system for grid stability. Nuclear Engineering and Design , 343 , 178–186. https://doi.org/10.1016/j.nucengdes.2019.01.010 .
Sawin, J. L. (2012). Renewables 2012-global status report . Paris: Renewable Energy Policy Network for the 21st Century.
Schill, W.-P., & Zerrahn, A. (2020). Flexible electricity use for heating in markets with renewable energy. Applied Energy , 266 , 114571. https://doi.org/10.1016/j.apenergy.2020.114571 .
Shayestegan, M., Shakeri, M., Abunima, H., Reza, S. M. S., Akhtaruzzaman, M., Bais, B., … Amin, N. (2018). An overview on prospects of new generation single-phase transformerless inverters for grid-connected photovoltaic (PV) systems. Renewable and Sustainable Energy Reviews , 82 , 515–530. https://doi.org/10.1016/j.rser.2017.09.055 .
Silva, N., Cunha, J. C., & Vieira, M. (2017). A field study on root cause analysis of defects in space software. Reliability Engineering & System Safety , 158 , 213–229. https://doi.org/10.1016/j.ress.2016.08.016 .
Sinsel, S. R., Riemke, R. L., & Hoffmann, V. H. (2020). Challenges and solution technologies for the integration of variable renewable energy sources—A review. Renewable Energy , 145 , 2271–2285. https://doi.org/10.1016/j.renene.2019.06.147 .
Soust-Verdaguer, B., Llatas, C., & García-Martínez, A. (2016). Simplification in life cycle assessment of single-family houses: A review of recent developments. Building and Environment , 103 , 215–227. https://doi.org/10.1016/j.buildenv.2016.04.014 .
Sovacool, B. K. (2009). The intermittency of wind, solar, and renewable electricity generators: Technical barrier or rhetorical excuse? Utilities Policy , 17 (3), 288–296. https://doi.org/10.1016/j.jup.2008.07.001 .
Spodniak, P., & Bertsch, V. (2020). Is flexible and dispatchable generation capacity rewarded in electricity futures markets? A multinational impact analysis. Energy , 196 , 117050. https://doi.org/10.1016/j.energy.2020.117050 .
Springer (2020). Publish and review Retrieved from https://www.springer.com/gp .
Stappel, M., Gerlach, A.-K., Scholz, A., & Pape, C. (2015). The European power system in 2030: Flexibility challenges and integration benefits. In An analysis with a focus on the pentalateral energy forum region agora Energiewende/Fraunhofer IWES Avaialble at http://www.agora-energiewende de Accessed Sept.
Suman, S. (2018). Hybrid nuclear-renewable energy systems: A review. Journal of Cleaner Production , 181 , 166–177. https://doi.org/10.1016/j.jclepro.2018.01.262 .
Taliotis, C., Taibi, E., Howells, M., Rogner, H., Bazilian, M., & Welsch, M. (2017). Renewable energy technology integration for the island of Cyprus: A cost-optimization approach. Energy , 137 , 31–41. https://doi.org/10.1016/j.energy.2017.07.015 .
Tareen, W. U., Mekhilef, S., Seyedmahmoudian, M., & Horan, B. (2017). Active power filter (APF) for mitigation of power quality issues in grid integration of wind and photovoltaic energy conversion system. Renewable and Sustainable Energy Reviews , 70 , 635–655. https://doi.org/10.1016/j.rser.2016.11.091 .
Telukunta, V., Pradhan, J., Agrawal, A., Singh, M., & Srivani, S. G. (2017). Protection challenges under bulk penetration of renewable energy resources in power systems: A review. CSEE Journal of Power and Energy Systems , 3 (4), 365–379.
Thellufsen, J. Z., Lund, H., Sorknæs, P., Østergaard, P. A., Chang, M., Drysdale, D., … Sperling, K. (2020). Smart energy cities in a 100% renewable energy context. Renewable and Sustainable Energy Reviews , 129 , 109922. https://doi.org/10.1016/j.rser.2020.109922 .
Twaha, S., & Ramli, M. A. M. (2018). A review of optimization approaches for hybrid distributed energy generation systems: Off-grid and grid-connected systems. Sustainable Cities and Society , 41 , 320–331. https://doi.org/10.1016/j.scs.2018.05.027 .
Ueckerdt, F., Hirth, L., Luderer, G., & Edenhofer, O. (2013). System LCOE: What are the costs of variable renewables? Energy , 63 , 61–75. https://doi.org/10.1016/j.energy.2013.10.072 .
Van Hulle, F., Holttinen, H., Kiviluoma, J., Faiella, M., Kreutzkamp, P., Cutululis, N., … Ernst, B. (2014). Grid support services by wind and solar PV: A review of system needs, technology options, economic benefits and suitable market mechanisms: Synthesis report of the REserviceS project .
Velasquez, M. A., Barreiro-Gomez, J., Quijano, N., Cadena, A. I., & Shahidehpour, M. (2019). Distributed model predictive control for economic dispatch of power systems with high penetration of renewable energy resources. International Journal of Electrical Power & Energy Systems , 113 , 607–617. https://doi.org/10.1016/j.ijepes.2019.05.044 .
von Meier, A. (2011). Integration of renewable generation in California: Coordination challenges in time and space. In 11th international conference on electrical power quality and utilisation , (pp. 1–6). https://doi.org/10.1109/EPQU.2011.6128888 .
von Meier, A. (2014). Challenges to the integration of renewable resources at high system penetration . Berkeley: California Institute for Energy and Environments. https://escholarship.org/uc/item/81x1c1t5 .
Wang, Y., Das, R., Putrus, G., & Kotter, R. (2020). Economic evaluation of photovoltaic and energy storage technologies for future domestic energy systems – A case study of the UK. Energy , 203 , 117826. https://doi.org/10.1016/j.energy.2020.117826 .
Wong, J., Lim, Y. S., Tang, J. H., & Morris, E. (2014). Grid-connected photovoltaic system in Malaysia: A review on voltage issues. Renewable and Sustainable Energy Reviews , 29 , 535–545. https://doi.org/10.1016/j.rser.2013.08.087 .
Worighi, I., Maach, A., Hafid, A., Hegazy, O., & Van Mierlo, J. (2019). Integrating renewable energy in smart grid system: Architecture, virtualization and analysis. Sustainable Energy, Grids and Networks , 18 , 100226. https://doi.org/10.1016/j.segan.2019.100226 .
Download references
Acknowledgements
This research supported by PNBP Universitas Syiah Kuala, Research Institutions and Community Service.
About the authors
Erdiwansyah: Born in Desa Meunafa Kec. Salang, Kab. Simeulue Aceh Province at 14 March 1984. Erdiwansyah is a lecturer at the Faculty of Engineering, University Serambi Mekkah, and Banda Aceh, Indonesia since 2014 until now. In 2020 this was registered as a PhD of Engineering Student at Universitas Syiah Kuala. The Master’s degree was pursued at the Department of Electrical Engineering at Universitas Syiah Kuala, Banda Aceh, Indonesia, completed in 2016. Furthermore, the bachelor’s degree was obtained in August 2012 from the Faculty of Engineering Department, Universitas Serambi Mekkah Banda Aceh. Currently, besides studying, he also helps research professors at Universitas Syiah Kuala, Banda Aceh.
Mahidin: Born in T. Gajah Kec. Tnh. Jambo Aye at 3 April 1970, the eldest one out of 6 siblings. Finished the elementary school in SDN Lhokbeuringen T. Gajah at 1982, Junior High School at SMPN 1 and Senior High School at SMAN 1 Panton Labu, Kec. Tnh. Jambo Aye, North Aceh, in 1985 and 1988, respectively. Moreover, undergraduate degree was earn at August 1994 from Department of Chemical Engineering, Syiah Kuala University. Magister degree was pursued at Department of Chemical Engineering, ITB in October 1999, and received Doctor of Engineering in Resource and Energy Science from Graduate School of Science and Technology, Kobe University in September 2003. He was awarded a professor in chemical engineering in 2018. Fields of research are treatment and utilization of energy resources, especially renewable energy resources and mix of energy (energy diversification).
Husni Husin Ph. D, is a Professor of Chemical Reaction Engineering at Syiah Kuala University. She joined Chemical Engineering Department since December 1994; Born: 1965, Samalanga, Aceh, Indonesia; Education: Syiah Kuala University (1990); Institute Technology Bandung (2000); National Taiwan University Science and Technology (NTUST) Taiwan (2011); The title of her dissertation is “Fabrication of La-doped NaTaO3 via H2O2 Assisted Sol-gel Route and Their Photocatalytic Activity for Hydrogen Production”; Her research interests are: Nanomaterial for Clean Energy production (Photocatalytic, Solar cell, Biodiesel, Biofuel, Fuel Cell), Heterogeneous Catalyst and Application, Adsorbent and Application;
Nasaruddin received the B.Eng. degree in Electrical Engineering from Sepuluh Nopember Institute of Technology, Surabaya, Indonesia in 1997. Then he received M. Eng and D. Eng in Physical Electronics and Informatics, Graduate School of Engineering, Osaka City University, Japan, in 2006 and 2009, respectively. He is a lecturer at Electrical Engineering Department, Syiah Kuala University. He was head of master of Electrical Engineering Programme; graduate school of Syiah Kuala University. Currently, he is head of Electrical and Computer Engineering Department, Faculty of Engineering, Syiah Kuala University. He has published several papers in international journals and accredited national journals. His research interests include digital communications, information theory, optical communications and ICT applications for disaster. He is a member of IEEE and IAES.
Dr. Ir. Muhammad Zaki, M. Sc is a lecturer and researcher in Chemical Engineering Department, Faculty of Engineering, Unsyiah since 1992. Received a Bachelor degree (Ir) in Chemical Engineering Department of Unsyiah, then continued S2 (M.Sc) and S3 (Dr.) at Universiti Kebangsaan Malaysia in Chemical and Process Engineering Department.
Muhibbuddin I completed my Ph. D in Technical and Vocational in Mechanical Engineering from The State University of Padang, Indonesia, in 2016 under the supervision of Prof. Dr. Nizwardi Jalinus and finished Master of Engineering degree in Mechanical Engineering Joint Programme between Gadjah Mada University and Bandung Technology Institute, in 2012. Since 2007 worked as Traineer Machining at Sandvik Light Industrial Park PT. Freeport Indonesia Tembagapura Papua Indonesia and resigned in 2008 for graduating as civil servant. Since college, I have been interested in Energy Conversion Machines especially water turbines, windmills and applied engineering. Besides studying, I am also active in Laboratory and Micro Hydro Power Plants Development Centers and research final project Bachelor; “Design and Manufacture of Transmission System a Portable Propeller Water Turbine 4 kW Capacity for Micro Hydro Power Plants”. The Master of Engineering focuses on the research; “Study of Utilization of Bamboo Parts as Blades of Pelton Water Turbine for Enhancing Rural Energy Technology to Support the Energy Independent Village Program”. Doctoral Research; “The development of Cooperative Project-Based Learning (CPBL) models for Energy Conversion Machines in Technical Vocational Education and Training in Mechanical Engineering”. I served as Head of Devision Human Resources Teacher and Education Personnel (Echelon III) Southwest Aceh Regency Education and Culture Office from 2018 to 2019. Since October 1, 2019 until now I am joined as a lecturer in Mechanical and Industrial Engineering, Faculty of Engineering, Syiah Kuala University, Banda Aceh.
The funding of this research is the grand research of the professor with the contract number of (32/UN11.2.1/PT.01.03/PNBP/2020).
Author information
Authors and affiliations.
Doctoral Program, School of Engineering, Universitas Syiah Kuala, Banda Aceh, 23111, Indonesia
Erdiwansyah
Faculty of Engineering, Universitas Serambi Mekkah, Banda Aceh, 23245, Indonesia
Department of Chemical Engineering, Universitas Syiah Kuala, Banda Aceh, 23111, Indonesia
Mahidin, H. Husin & M. Zaki
Department of Electrical and Computer Engineering, Universitas Syiah Kuala, Banda Aceh, 23111, Indonesia
Department of Mechanical and Industrial Engineering, Universitas Syiah Kuala, Banda Aceh, 23111, Indonesia
Muhibbuddin
You can also search for this author in PubMed Google Scholar
Contributions
1. Erdiwansyah: The first author acts as the author of all article content and data collection such as literature searches and other data that support this research. 2. Mahidin: The second author acts as the draft writer of the article and also as a review for the refinement of the article before it is sent to this journal. 3. H. Husin: The third author acts as a controller of the writing done by the first author. In addition, the third author is also tasked with analyzing the literature data collected and written by the first author. 4. Nasaruddin: The fourth author acts as a drafter and design of articles written by the first author. In addition, the fourth author is also a policy maker for this article and serves as the final review and editing of this journal. 5. M. Zaki: The fourth author acts as a contributor to research funding in addition to funding from the grand research. The fourth author also acts as analysis and refinement of the final article. 6. Muhibbuddin: This sixth author acts as a fund contributor for checking language and words and sentences for English language experts. The sixth author has also helped to revise the end of the journal jointly with all the authors in this article. The author(s) read and approved the final manuscript.
Corresponding authors
Correspondence to Erdiwansyah or Mahidin .
Ethics declarations
Competing interests.
There are no conflicts to declare.
Rights and permissions
Open Access This article is licensed under a Creative Commons Attribution 4.0 International License, which permits use, sharing, adaptation, distribution and reproduction in any medium or format, as long as you give appropriate credit to the original author(s) and the source, provide a link to the Creative Commons licence, and indicate if changes were made. The images or other third party material in this article are included in the article's Creative Commons licence, unless indicated otherwise in a credit line to the material. If material is not included in the article's Creative Commons licence and your intended use is not permitted by statutory regulation or exceeds the permitted use, you will need to obtain permission directly from the copyright holder. To view a copy of this licence, visit http://creativecommons.org/licenses/by/4.0/ .
Reprints and permissions
About this article
Cite this article.
Erdiwansyah, Mahidin, Husin, H. et al. A critical review of the integration of renewable energy sources with various technologies. Prot Control Mod Power Syst 6 , 3 (2021). https://doi.org/10.1186/s41601-021-00181-3
Download citation
Received : 13 July 2020
Accepted : 12 January 2021
Published : 23 February 2021
DOI : https://doi.org/10.1186/s41601-021-00181-3
Share this article
Anyone you share the following link with will be able to read this content:
Sorry, a shareable link is not currently available for this article.
Provided by the Springer Nature SharedIt content-sharing initiative
- Integration RE
- Energy source
- Technology system energy
- Power system
- Variable RE
- Open access
- Published: 27 August 2024
Virtual power plants: an in-depth analysis of their advancements and importance as crucial players in modern power systems
- Sobhy Abdelkader 1 , 2 ,
- Jeremiah Amissah 1 &
- Omar Abdel-Rahim 1 , 3
Energy, Sustainability and Society volume 14 , Article number: 52 ( 2024 ) Cite this article
Metrics details
Virtual power plants (VPPs) represent a pivotal evolution in power system management, offering dynamic solutions to the challenges of renewable energy integration, grid stability, and demand-side management. Originally conceived as a concept to aggregate small-scale distributed energy resources, VPPs have evolved into sophisticated enablers of diverse energy assets, including solar panels, wind turbines, battery storage systems, and demand response units. This review article explores the evolution of VPPs and their pivotal roles as major stakeholders within contemporary power systems. The review opens with a definition of VPPs that clarifies both their fundamental traits and technological foundations. A historical examination of their development highlights major turning points and milestones that illustrate their transforming journey.
The methodology used for this article entailed a thorough examination to identify relevant studies, articles, and scholarly works related to virtual power plants. Academic databases were used to gather relevant literature. The literature was organized into categories helping to structure and present information in a logical flow based on the outline created for the review article . The discussions in the article show that the various functions that VPPs perform in power systems are of major interest. VPPs promote the seamless integration of renewable energy sources and provide optimum grid management by aggregating distributed energy resources, which improves sustainability. One of the important components of this evaluation involves taking market and policy considerations. Examining worldwide market patterns and forecasts reveals that VPP usage is rising, and that regulatory frameworks and incentives have a bigger impact on how well they integrate.
Overcoming obstacles is a necessary step towards realizing full VPP potential. For VPPs to be widely adopted, it is still essential to address technological and operational challenges as they arise. Diverse stakeholders must work together to overcome market obstacles and promote the expansion of the VPP market. This analysis highlights the potential for VPPs to propel the evolution of contemporary power systems toward a more sustainable and effective future by highlighting areas for future research and development.
There is an urgent need for creative and sustainable alternatives as the world’s need for energy rises, while fossil fuel-based power generation methods are increasingly scrutinized for their environmental effects [ 1 ]. Centralized alternating current power networks have been widely installed and used worldwide since the 1880s. Evaluations from the 2023 statistical global energy review [ 2 ] revealed that about 82% of the world’s primary energy source comes from fossil fuels like coal oil, and natural gas but their utilization produces greenhouse gas emissions that harm the environment and cause climate warming which has triggered the current global climate crisis [ 3 ]. The contribution of the different sources to world energy consumption is shown in Fig. 1 .
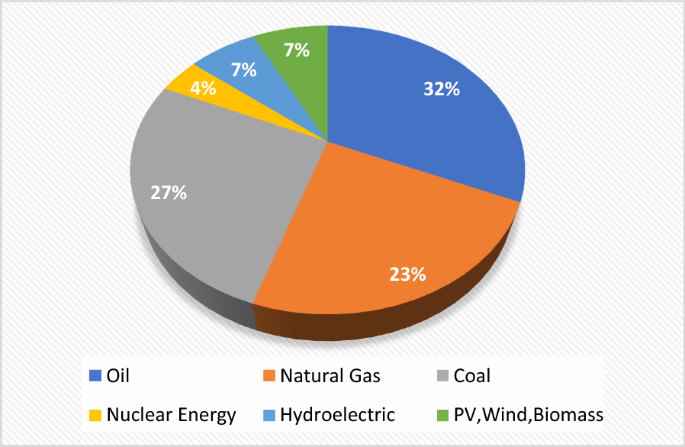
Global energy sources data
On the other hand, energy demand has grown significantly as a result of global economic growth. The demand for electricity has increased steadily over the past decades, by an average of 15%, and is anticipated to increase by 30% by 2040 [ 4 ]. This calls for innovative ideas to support the demand while looking out for the environment. Therefore, distributed energy resources (DERs) must be considered to lessen the detrimental environmental impacts of fossil fuels [ 1 ]. DERs are decentralized energy systems that produce, consume and store energy and are preferably located close to where electricity is consumed. These resources include batteries, wind turbines, solar panels, etc. DERs have been integrated in the power system networks (PSN) and have reduced the effects of energy generation from fossil fuels, furnishing stakeholders with economic and technical benefits [ 5 ]. While DERs offer power systems opportunities, they also bring with them challenges because of their intermittent and stochastic nature. DERs are often described as stochastic and intermittent due to their inherent characteristics and the factors that influence their generation. This nature of DERs is caused by elements including weather changes, operational uncertainties like maintenance, and equipment performance, which can result in unanticipated variations in DER generated or connected output. Instability in the grid is brought on by the rising use of DERs on the demand side, which worsens load demand fluctuations. As a result, real-time monitoring and dispatching are essential for the grid’s safe operation [ 6 , 7 , 8 , 9 ]. Furthermore, the power system needs more adaptability, which can be provided by several mechanisms, such as demand-side management, and energy storage systems (ESS). The only way to properly use these sources to increase their grid contributions is through optimal coordination between different agents [ 10 ].
Over the years, various research has been conducted to address the above challenges and many solutions have been proposed. VPPs have emerged as a ground-breaking solution in an era of energy transition and growing emphasis on sustainable power generation, altering the landscape of contemporary power systems [ 11 ]. VPPs have evolved as key players in promoting efficiency, flexibility, and resilience in the energy industry thanks to their capacity to integrate a variety of energy supplies and improve grid management [ 12 , 13 ].
A VPP is an energy management system that aggregates and coordinates diverse array of DERs, including photovoltaics, wind turbines, battery energy storage systems (BESS), and demand response technologies. The primary function of a VPP is to optimize the collection of these DERs in response to grid conditions, energy demand, and market signal. Through advanced control algorithms and real-time monitoring capabilities, VPPs dynamically adjust energy dispatch schedules, balances supply and demand, and enhance grid stability and reliability.
It is important to note that the concept of VPPs shares some basic similarities with that of the smart grid. However, unlike the VPP which focuses on the aggregation and optimization of DERs, smart grid, on the other hand, encompasses a broader range of functionalities aimed at modernizing the entire electricity supply chain. It can be said that the VPP augment the operation of the smart grid by providing ancillary support like supply and demand balancing to the smart grid.
The combination of these various resources enables the VPP to function as a cohesive and adaptable entity, to be able to react in real-time to grid signals and market conditions [ 14 , 15 ]. In the late 1990s, a pioneering shift in energy research and innovation emerged with the exploration of aggregating distributed resources into a unified virtual power entity, laying the groundwork for the conceptualization and development of VPPs [ 13 ]. Since then, VPPs have evolved from theoretical notions to real-world applications owing to technical developments, and breakthroughs in communication technology. The adoption of VPPs has been hastened by the spread of smart grid technologies and the rise of renewable energy resources (RERs), making them a crucial component of contemporary power systems [ 12 , 16 ].
It is impossible to overstate the importance of VPPs as significant participants in contemporary power systems. VPPs are essential for facilitating the seamless integration of intermittent renewable resources into power grids as they shift from fossil fuel-based generation to renewable-dominated systems [ 3 , 17 , 18 ]. In addition, VPPs can control electricity consumption patterns to correspond with variations in renewable generation. Demand-side management improves grid reliability and efficiency by lowering peak demand and reducing grid congestion [ 19 , 20 ]. VPPs also significantly contribute to the optimization of the energy market. VPPs are crucial actors in the developing electricity market because of their involvement in energy trading and the provision of ancillary services, which help to stabilize prices and maintain system resilience [ 11 , 21 ]. A typical architecture of a VPP is shown in Fig. 2 . With the aid of technology like cloud computing, a VPP aggregates various power consumers, ESS, and power generators to provide flexible adjustments. A communication protocol is used by the components of a VPP to transfer data to the VPP communication system. This communication protocol enables efficient coordination for the VPP to adjust energy production which allows supply to the grid with dependable cost-effective electricity via the electricity market [ 22 ]. The data acquisition platform aids in gathering information about the generation, consumption, and state of charge of the portfolio of DERs for optimal decision-making.

Architecture of a VPP
From the above discussion, it is clear that VPPs have become an important player in modern power systems, providing a dynamic and revolutionary method of managing energy. The idea of VPPs has recently received a lot of interest in energy systems. Studies have provided insightful information by highlighting their potential to transform the way we produce, distribute, and use power. It is critical to understand that this dynamic and developing discipline poses several notable issues, gaps, and areas that require added research.
In the review presented in [ 23 ], an overview of VPP operations, including the integration of DERs, controlled loads, and EVs for resource aggregation and cooperative optimization as well as market and grid operations, is the goal. The evaluation did however not discuss regulatory and policy issues that might affect how widely VPPs are used and implemented in the power market.
Also, the difficulties, solutions, and prospects related to the conceptual review of the conversion of a microgrid to a VPP have also been covered by [ 24 ]. The overview examines RERs integration, opportunities for VPPs in the field of smart distribution systems, and effective management mechanisms. The management mechanism, however, did not discuss the optimization of the DERs for optimal operation. Authors in [ 25 ] gave a thorough overview of the VPP concept and its potential advantages in integrating DERs to assist grid security and stability. Resource optimization, as a main part of the VPP operation, is not covered in this study. Also, Ref. [ 11 ] provided an overview of VPP models and how they interacted with various energy markets. Finding the most profitable VPP scheme to be implemented in each regulatory environment is the focus. DER integration challenges, which affect the operation of VPPs in the energy markets, are not considered in this study. In [ 26 ], the idea of VPPs to participate in various energy markets is proposed. The model evaluates the VPP's technical and commercial prospects. Engaging in various energy markets revolves around sharing of data between the VPP and operators of the markets. The issue of data privacy and cybersecurity was not included in this study. Authors in [ 27 ] provided a review with a focus on integrating DERs into the electricity grid. The assessment gave a summary of the development and use of VPP for carbon reduction in the Chinese power system. The study, however, did not cover technologies that can improve the management and operation of VPPs, notably in addressing the intermittent and volatile nature of DERs. In the domain of energy management, authors of [ 28 ] provided a summary of resource scheduling in VPPs and addressed questions on scheduling procedures. However, despite concentrating on both technical and economic elements of scheduling in VPPs, this analysis did not address potential influences like the state of the energy markets that could have an impact on the scheduling issue. The case of a multi-energy coupled VPP has been presented in [ 29 ]. The purpose of this study was to address the advantages of multi-energy linked VPPs engaging in various energy markets. The issue of enhanced communication technology, data privacy and cybersecurity are some of the challenges which were not featured in this study.
The idea and structure of VPPs are concisely described in [ 30 ] with regard to its two main goals—energy management and power markets. Solutions are suggested to alleviate the problems with DER uncertainties that were highlighted. In order to create future sustainable power grids, authors of [ 3 ] have presented a comprehensive overview of the cutting-edge VPP technology. The study discusses recent technological advancements as well as the significant economic benefits of VPPs. However, this study did not cover the legislation that specifies how VPPs can access and participate in the energy markets. Below are some of the gaps found in existing literature:
Analysis of cybersecurity and data privacy as crucial elements in the VPP development.
Environmental and sustainability focus. The SDGs that VPPs could support, and how the support can be achieved.
Rigor analysis of legislation or regulations which will dictate the operation of the VPP.
Considering the above research gaps in literature, this review article advances the knowledge of energy systems by providing a thorough analysis of VPPs, their historical development, and their crucial roles as essential stakeholders in modern power systems. There will be focus on technical and market operations, real-world case studies, the identification of challenges and prospects, the emphasis on technical and market operations highlight the relevance and transformative potential of VPPs in creating sustainable and effective energy ecosystems. The contributions of this paper can be summarized as follows:
Comprehensive understanding of VPPs to provide readers with a concise definition, key traits, and core values of VPPs.
Tracing historical developments of VPPs from their theoretical roots to their current popularity.
Emphasis on VPPs as key stakeholders in modern power systems. This emphasis highlights the vital role that VPPs play in ensuring grid stability, fostering the integration of RES, and promoting sustainability.
Integration of technical and market aspects by providing a comprehensive analysis of VPP operation. This integration is crucial as it shows that VPPs actively participate in energy markets and actively optimize energy resources, which facilitates effective electricity trading and grid balancing.
Application of cybersecurity and data privacy techniques that protect the VPP from cyber threats, assuring grid stability, data integrity, and consumer trust in the ever-changing energy sector.
Real-world case studies of VPP deployments to offer insights and experiences.
Discussion of the regulatory frameworks that control how VPP operates.
Identification of challenges, providing recommendations, and prospects.
VPP advancements
The traditional centralized power generation model is being replaced by a decentralized, adaptable, and sustainable system thanks to VPP, which represents a revolutionary paradigm in the energy sector. Early theoretical ideas from the late twentieth century established the foundation for the development of VPPs and their eventual prominence in modern power systems [ 31 , 32 ]. This part of the paper will focus on the evolutionary journey of VPPs, highlighting the early concepts, key milestones, and technological advancements that shaped their development into critical enablers of modern energy ecosystems.
The embryonic stage (1990s–2000s)
Although the idea of VPPs was initially put forth in the 1997 [ 13 ] by Dr. Shimon Awerbuch, it did not really take off until the early 2000s. Early academic publications proposed the idea of coordinating and optimizing a portfolio of distributed energy resources to increase operational effectiveness and grid reliability. However, due to limited technological capabilities and a lack of enabling legal frameworks, the practical deployment of VPPs remained primarily theoretical at this point. Also, the absence of developed distributed generating technology, the high cost of communication and control systems, and the regulatory uncertainties surrounding VPPs were some of the causes of lack of practical deployment. References [ 33 , 34 , 35 , 36 , 37 , 38 ] provides a description of the early years concept of the VPP, its difficulties, including consumer resistance to participating, economic viability in infrastructure setup, investors' perceptions of risk, and grid operators' reluctance to adopt the unique strategy.
The breakthrough stage (2010s–2020)
The growth years presented milestones and key turning points in VPP deployment from the early years. At this point, the VPP has encountered rapid growth as a result of increasing interest in adoption of distributed generation technology, decreasing communication and control system costs, and expanding regulatory backing for VPPs. In a declaration on the future of the European electricity market that was issued in 2011, the European Commission emphasized the potential of VPPs to increase grid flexibility and integrate renewable energy. This communication aided in increasing policymakers’ and stakeholders’ understanding of VPPs [ 39 , 40 , 41 , 42 , 43 , 44 , 45 ]. Later, in March 2023, it was amended in Strasbourg, France, by recommending an expansion of the EU electricity market structure to further integrate RESs, improve customer protection and industrial competitiveness [ 46 ]. Notable milestones of the growth years include grid integration [ 47 ], market participation [ 48 ], technological advancement, and demand response programs[ 49 ], allowing aggregated DERs to respond to grid signals and enhance grid stability [ 50 ]. This marked the initial practical application of VPPs, showcasing their ability to support grid operations.

The future (2021 and beyond)
The demand for flexible grids and the incorporation of RESs is anticipated to drive further growth of VPP. VPPs are viewed as one of the techniques to lower carbon emissions and increase energy efficiency [ 51 ]. The key drivers for this growth are the increasing deployment of distributed generation technologies (DGT), falling cost of communication and control systems, growing regulatory support for VPPs, and also prosumers who want to receive incentives for their surplus generation [ 45 ].
In summary, it is evident that early theoretical insights were followed by practical and revolutionary applications in modern power systems as VPPs evolved. The development of VPPs into essential enablers of decentralized, flexible, and sustainable energy ecosystems has been shaped by significant turning points and milestones, as well as technological development and innovations. A thorough summary is provided in Table 1 for further reading.
VPP planning, roles, and sustainability
VPP planning is a crucial and multifaceted process that entails strategic design, coordination, and optimization to provide effective and dependable energy management. The main goal of VPP planning is to maximize the advantages for both grid operators and consumers while optimizing the potential of varied DERs and guaranteeing their seamless integration with the power grid. The planning approach necessitates a thorough comprehension of the energy landscape, individual DER capabilities, market dynamics, and regulatory frameworks.
To ensure that VPPs perform as planned and expected, their technological constraints must be recognized and measured [ 55 ]. Before interacting with external and internal elements, the VPP schedules and plans its operations. It is also a good performance criterion for the VPP to keep accurate data to engage the electricity market and reap favorable effects by analyzing the uncertainties resulting from elements like weather and producing forecasts with a high level of assertiveness [ 56 ]. The issue of forecasting will be discussed later in the section dedicated to the roles of VPPs. The VPP operations may be constrained by infrastructure, technological, and technical limits [ 57 ]. The model shown in [ 26 ] emphasizes the importance of effectively measuring and managing controllable loads in heating, ventilation, and air conditioning (HVAC) systems. Also, it emphasizes the significance of photovoltaic (PV) and BESS influences in determining the viability and adaptability of a VPP. VPPs can improve their coordination with all stakeholders by developing a methodical technique for evaluating and controlling power availability at time intervals. Surely, this enhances the performance of the VPP and enables a more seamless interaction with the power grid.
VPP planning also includes economic and legal factors in addition to the technical ones. The aspects of technical and economic frameworks of the VPP will be delved deeper in the sections dedicated to the technical and economic aspects of VPPs. It is important to note that good operational planning directly affects good economic outcomes [ 55 ]. The economic viability of the VPP and its prospective revenue streams, including energy trading [ 58 ], demand response participation [ 59 ], and the supply of ancillary services [ 21 ], are assessed using financial models and cost–benefit analysis [ 60 ]. Collaboration with grid operators, legislators, and other stakeholders is also necessary for successful VPP planning to overcome regulatory obstacles and build an environment that facilitates VPP integration. To ensure effective planning, the VPP should be continuously monitored and improved to respond to shifting grid conditions and market dynamics [ 61 ]:
VPP planning opens the way for a more resilient, and sustainable energy future by integrating technological, economic, and regulatory factors. It has enormous potential to optimize resource use, improve grid stability, and contribute to the global quest for a reduction in carbon emissions produced by energy systems. It is therefore imperative that stakeholders comprehend the complexities of VPP planning to influence the energy industry’s future and advance the cause for greener and a more sustainable and effective energy future. This planning phase can be summarized as: aggregating existing and new energy resources.
Ownership structure: The internal ownership structure of VPPs can vary depending on the specific implementation and stakeholders involved. It may involve collaboration between multiple stakeholders including energy producers, consumers, and aggregators.
Regulating and market considerations governing energy markets and grid operations.
Implementation of an energy management system to provide functionalities such as real-time monitoring, forecasting, dispatching, and scheduling energy resources to meet grid requirements and maximize economic benefits.
Agreement formulation such as power purchase agreements.
Profit sharing mechanisms taking into consideration factors such as investment contributions, operational cost, risk allocation, etc.
Compensation structures for various stakeholders involved in the VPP including incentives for demand response participations from consumers.
The way electricity is produced, controlled, and used has been revolutionized by VPPs as explained in the previous sections. VPPs are flexible and dynamic entities that perform a variety of roles in modern power systems. Because of the variety and importance of their tasks, they are key players in creating an energy ecosystem that is sustainable, effective, and resilient. The following are the main responsibilities of VPPs in power systems.
Aggregation of DERs: Various DERs, such as solar panels, wind turbines, ESS, EVs, and demand response loads are gathered by VPPs. VPPs construct an adaptable and manageable portfolio of assets by combining these decentralized resources into a single virtual entity. Through this aggregation, grid management is improved, enabling the VPP to maximize DER usage in response to grid signals. The DERs’ activity within the VPP is managed and coordinated by the VPP operators. The main responsibility is resource optimization and involvement in energy markets.
The authors of [ 62 ] described the aggregator concept as a central control node that collects information from both the power grid and controlled loads. A load aggregator can also serve as a conduit between the controllable loads and the grid operator, allowing the regulated management to consider user and grid benefits simultaneously. When interfacing with the power market, aggregators are employed in power charging models for EVs to help optimize the batteries’ charging as well as the modeling of driving patterns and price estimates [ 63 ]. As DERs are dispatched depending on compensation rates and power levels, an aggregator can stand in for them to maximize profits [ 64 ]. Furthermore, in [ 65 ], for a power market with bilateral contracts, the aggregator has the facility to select between various power plants based on power-cost-based offers.
Grid stabilization and reliability: VPPs make a major contribution to the reliability and stability of the grid. VPPs maintain a stable and steady supply of electricity while minimizing the possibility of blackouts and voltage variations by balancing energy generation and consumption from various DERs [ 66 ]. They are able to provide ancillary services like frequency regulation and voltage management, which are essential for preserving grid stability [ 67 ]. The general stability and dependability of the electrical system are the responsibility of grid operators. In accordance with grid norms and standards, the grid operators work with VPP operators to incorporate DERs.
Renewable energy integration: In 2016, in Paris, an emission reduction plan was enacted which has made the use of DERs very essential [ 68 , 69 , 70 ]. This integration is the VPP operator’s responsibility. This is accomplished by coordinating the operation of diverse RERs, such as solar panels, wind turbines, and such that they work as a unified system. However, due to their erratic nature, integrating RESs into the power systems presents its own challenges [ 71 , 72 ]. These challenges come about because of generation fluctuations due to weather conditions and time of the day. The variability adds complexity to power system operations. For instance, rapid changes in wind speed or cloud cover can result in fluctuations in generation, requiring grid operators to make quick adjustments to maintain system stability. VPPs take on this problem by combining several RESs and using intelligent management processes, they make it easier for the integration of the RESs effectively. They ensure the integration of these RESs to provide a steady supply of electricity while lowering reliance on conventional fossil fuel-based power plants.
Authors in [ 72 ] proposed a solution for integration of RESs into the grid to maintain power quality. This is important because RESs are becoming increasingly popular due to their environmental benefits, but they can also introduce power quality issues. This is a challenge that a VPP is sought to address. Large scale penetration of RESs means a hike in capital and operational cost. Authors in [ 73 ] discussed a mechanism that could aid in lowering the high cost of RESs integration and bringing electricity prices into affordable band. Spreading the benefits of renewable integration into the spheres of agriculture, where in [ 74 ], authors have created a mechanism to encourage energy-efficient agriculture by minimizing dependency on fossil fuels for water-table pumping. Through the aggregation and optimization of DERs, VPPs enable farmers to reduce their dependency on fossil fuels while enhancing energy efficiency and resilience in agricultural practices. This synergy not only fosters economic sustainability for farmers, but also contributes to the broader goal of renewable energy integration, paving the way for a greener energy future.
Successful integration depends on several important aspects. Forecasting methods that accurately estimate the patterns of RESs generation must be put in place [ 75 , 76 ]. This allows better grid management and optimization of the DERs. The VPP employs such tools to better manage the generation of DERs. A summary of various forecasting techniques provided in the literature is listed in Table 2 . Analysis of forecasting models to aid in the integration of RESs in the context of VPPs has been provided in [ 77 ].
Moreover, for optimal integration of RESs, the power grid must be modernized with smart technologies. Real-time monitoring, control, and communication between DERs and grid infrastructure are made possible using smart approaches like the VPP [ 16 , 78 , 79 ]. This improves the reliability and effectiveness of the grid. Additionally, VPPs provide beneficial grid functions, such as frequency regulation [ 67 ] and voltage control [ 80 ] in addition to balancing energy supply and demand [ 81 ]. These services boost the grid’s dependability and resilience even more, promoting a stronger energy infrastructure that can handle the rising proportion of RESs.
The VPP approach to integrating RESs into the power grid is a cutting-edge strategy that is revolutionizing the way energy is produced, distributed, and consumed. VPPs offer an effective response to the problems caused by intermittent renewables by utilizing the combined potential of DERs and modern technology. VPPs will unquestionably be essential in advancing the transition to a cleaner, more dependable, and efficient energy system as the world progresses toward a sustainable energy future.
DER technologies applied in VPPs
In VPPs, various DERs are used, including solar panels, wind turbines, ESS, EVs, and demand response loads. These DERs are aggregated and optimized within the VPPs, allowing for efficient management and coordination [ 55 ]. By harnessing the collective capacity of diverse DERs, VPPs enhance grid stability, enable renewable energy integration, and support demand response strategies, contributing to a more sustainable and flexible energy ecosystem. A VPP should ensure that DER integration keeps the system operating properly by ensuring the stakeholders’ continual consumption requirements [ 92 ]. Various DER technologies applied in VPPs in the reviewed literature are summarized in Table 3 .
Out of the 15 References evaluated regarding DER technologies used in VPPs, it is evident from Table 3 that wind turbines and solar panels hold the largest share, as shown in Fig. 3 . It proves how easily the technology of wind turbines and solar panels have been embraced. However, more renewables should be added to the energy mix to hasten the shift to a less carbon-oriented energy landscape.
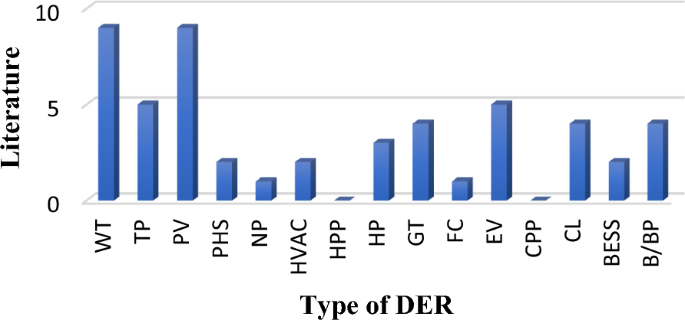
DER application in literature
VPP sustainability focus
One of the viable ways to address numerous Sustainable Development Goals (SDGs) of the United Nations (UN) and contribute to a more sustainable energy future is through VPPs. By encouraging the integration of RESs and boosting energy efficiency, VPPs, as a fundamental enabler of the energy transition, contribute significantly to achieving SDG 7 (Affordable and Clean Energy). VPPs promote the integration of sustainable energy into the power grid by aggregating and optimizing DERs thereby lowering greenhouse gas emissions and addressing climate change (SDG 13—Climate Action).
Additionally, through promoting technological advancements and innovation in the energy industry, VPPs provide a substantial contribution to SDG 9 (Industry, Innovation, and Infrastructure). VPPs promote grid modernization and improve overall energy infrastructure by integrating smart grid technologies, advanced analytics, and artificial intelligence. These developments result in more effective and adaptable energy systems, advancing the objectives of SDG 9 to develop robust infrastructure and encourage sustainable industrialization.
However, while VPPs offer considerable potential for achieving various SDGs, several challenges must be addressed to ensure their long-term sustainability. Access to VPP technologies must be equally available, as this can influence SDG 1 (No Poverty) and SDG 10 (Reduced Inequalities). For VPPs to be deployed in a way that supports SDG goals for eradicating poverty and minimizing inequality, marginalized people and neglected areas must be able to benefit from them. In simple terms, it is essential to make sure that everyone has an equal opportunity to profit from VPPs to realize SDG 1 and SDG 10. This calls for figuring out ways to make technology more accessible and inexpensive for everyone, especially those living in rural or underdeveloped areas. By doing this, VPPs may contribute to the development of a more just and sustainable energy future in which everyone, regardless of financial situation, has access to safe and dependable energy.
Furthermore, the environmental impact of VPPs [ 108 ] and their associated technologies require careful consideration to achieve SDG 12 (Responsible Consumption and Production). Lithium-ion batteries, which are used in ESS, are one example of a crucial mineral and material whose demand is on the rise, prompting questions regarding responsible sourcing, recycling, and end-of-life management. It is not a surprise that there has been extensive literature on ways to increase the lifespan of lithium-ion batteries [ 109 ]. Authors in [ 110 ] proposed a precise lifespan model for the battery cells used in VPP applications. To reduce the negative environmental and social effects of VPP deployment, sustainable methods must be implemented in material sourcing and VPP operation.
Moreover, numerous steps can be taken to guarantee the sustainability of a VPP itself. Stakeholders must work together to build supporting regulatory frameworks and financial incentives for VPP development. VPPs will become more widely available and long-lasting if investments are encouraged in their research, development, and implementation. This will also encourage technological breakthroughs and cost reductions. Also, a successful integration of VPPs into the energy economy depends on raising consumer awareness and engagement. The acceptance of VPP technology can be increased by educating consumers about the advantages of VPP participation, such as lower energy costs and increased grid reliability [ 111 , 112 ].
To sum up, VPPs have a significant potential to help achieve several SDGs pertaining to renewable energy, tackling climate change, and sustainable infrastructure. They support SDGs 7 and 9 by fostering the integration of RESs and improving energy efficiency. To achieve more general sustainability goals, it is necessary to address issues with fair access to VPP advantages and responsible use and production. VPPs are critical enablers of a greener, more inclusive, and resilient energy future and can help accomplish specific SDGs by establishing supportive policies, encouraging innovation and consumer engagement. Using VPP's revolutionary potential in promoting the UN’s sustainability agenda [ 113 ] requires advocating for and making contributions to their sustainable deployment and optimization.
Cybersecurity and data privacy
The protection of the grid’s stability and dependability is one of the main justifications for prioritizing cybersecurity in VPP application. As crucial nodes in the grid, VPPs coordinate the functioning of DERs and provide a constant and reliable supply of electricity. A cyber-attack on a VPP has the potential to impair energy production, distribution, and grid management, resulting in power outages [ 114 ] and large financial losses.
The efficient operation of VPPs depends on data integrity [ 115 ]. For making decisions about the generation, distribution, and use of energy, VPPs depend on accurate data. Cybersecurity measures guard against data alteration or manipulation, ensuring that VPP operators have reliable data for maximizing energy resources and delivering crucial grid services. In order to increase consumer and prosumer confidence in VPP services, data privacy procedures on data collection and usage are essential [ 116 ].
VPPs are desirable targets for cybercriminals because of their crucial functions in grid management and their strength in the marketplace. VPPs are shielded by cybersecurity from a variety of dangers, such as malware and hacker attempts [ 117 ]. To address the cybersecurity issues, various approaches have been suggested and has been categorized by [ 118 ] as human and non-human approaches. Human approaches like updates and incremental patches installation aids in robust security posture, addressing vulnerabilities in software, but also require reboots causing downtime to regular operations. Engaging in customer interactions also creates awareness to recognize and respond to potential threats. However, allocating time and resources may be challenging for organizations with limited budgets and manpower.
Non-human approaches like the adoption of blockchain technology reduce the risk of single point failure as the technology operates on a decentralized network. This enhances resilience, making it more challenging for attackers to compromise the entire system. Another non-human approach is cloud computing which typically encrypts data during transmission and storage. This safeguards sensitive information from interception or unauthorized access.
Data privacy and cybersecurity are essential elements of VPP operations. They protect against cyberthreats, guarantee data integrity, enhance grid stability [ 119 ], promote consumer trust, enable regulatory compliance, and support the viability of VPPs financially. To ensure a secure, dependable, and sustainable energy future, cybersecurity and data privacy must be prioritized as VPPs continue to develop and broaden their role in contemporary energy systems [ 120 ].
Regulation and compliance
The operation of VPPs is greatly influenced by legislative or regulatory activities. This section will cover the regulatory structure that governs VPPs, emphasizing significant importance and their effects on the energy industry.
In the domain of grid integration standard and requirements, regulating bodies establish grid codes and integration standards that the VPP must adhere to when connecting to the electrical grid. The safe and dependable grid integration of DERs is ensured by these standards. The basis for secure VPP functioning is grid codes and standards. A manual for connecting DERs to the utility grid is provided by the IEC 62786. DER planning, operation, protection, and connectivity to distribution networks are the key applications. A global agreement on the use of DER in electrical power systems is being sought through the IEEE 1547 set of standards. This standard has received widespread acceptance on a global scale in outlining the requirements for the design, implementation, testing, and security of all sorts of DERs. Due to the increased penetration of DERs and the need to maintain system stability, the IEEE 1547 has recently been updated to IEEE 1547-2018 and IEEE 1547.1-2020 [ 121 ]. A crucial series of standards released to control the grid’s interconnection and operability is the IEEE 2030. It is modified to implement cutting-edge communication and information technologies that provide interoperability solutions for the promotion of DER connectivity.
The European Committee for Electrotechnical Standardization (CENLEC), which is made up of 34 European Nations, oversees standardization efforts to increase commercial viability and foster technological growth. The CENLEC released the EN 50549-1 and EN 50549-2 DER integration standards with the goal of addressing all DER capabilities that are necessary for operation in tandem with distribution networks [ 121 ].
Also, there may be regional variations in regulations governing the integration of DERs with the grid [ 121 ]. For example, Canadian standards C22.3 No. 9 and C22.2 No. 257 offer technical advice for DER integration with the grid at medium and low voltage under 50 kV and low voltage systems under 0.6 kV, respectively. The British standard BS EN50438:2007 also offers technical advice for DER interconnection. The VDE-AR-N 4105 standard in Germany also offers technical recommendations for connecting DERs and low voltage systems. The JEAG.9701-2001 standard in Japan offers technical recommendations for distributed generating grid-connection. The standard permits DER owners to sell surplus energy to utility grids and mandates that power grids supply DER owners with backup power.
Various environmental and sustainability regulations may pertain to different jurisdictions [ 122 ], and they may provide incentives or requirements for VPPs to assist the integration of RERs and the reduction of emissions. In certain regions, these rules may have an impact on how VPPs function. The level of support for VPPs that use RERs may vary depending on the targets and incentives that jurisdictions set for renewable energy [ 123 ].
VPP operators and stakeholders must negotiate a complicated regulatory environment that is unique to their locations. It is essential for the implementation and operation of VPPs to comprehend and follow local legislations. Furthermore, as VPPs become more crucial to the world’s energy landscape, regulators and industry participants must cooperate to unify rules and encourage uniformity in grid integration techniques across various jurisdictions.
Technical aspects of VPPs
The technical operations of a VPP involve a series of complex and coordinated processes to efficiently manage and optimize the aggregated DERs within the VPP. According to Ref. [ 124 ], the technical features of VPPs provide dynamic interaction for the integration of power distribution based on auxiliary services. These technical operations can vary depending on the specific architecture and goals of the respective VPP. This section of the paper delves into the technical intricacies of VPPs and explores their roles as key enablers in the transition toward a sustainable and resilient energy future. Some of these technical aspects of the VPPs are emphasized below:
Resource optimization and scheduling: In a VPP, resource optimization and scheduling of various DERS are essential to achieve efficient and reliable energy management [ 28 , 125 ]. It is also important to note that advanced algorithms and real-time data analytics [ 76 ] as summarized earlier in Table 2 are employed to forecast energy generation and demand profiles, ensuring dynamic resource optimization. The VPP intelligently dispatches DERs based on grid conditions and market signals, balancing supply and demand to enhance grid stability and maximize revenue generation [ 126 ]. By coordinating diverse DERs, VPPs optimize energy use, contribute to renewable integration, and support grid flexibility, making them crucial enablers in the transition to a sustainable resilient energy ecosystem.
A summary of the relevant literature in accordance with resource optimization and scheduling is provided in Table 4 .
Load balancing and grid support/ancillary service: The load balancing and grid support functions of a VPP are very crucial [ 135 ]. The VPP dynamically modifies energy generation and consumption to fit grid demands by aggregating and optimizing various DERs. While storing excess energy during times of low demand, the VPP can supply additional power from DERs during times of peak demand to balance out high demand. This load-balancing ability makes VPPs essential for guaranteeing a dependable and resilient electricity supply since it improves grid stability, lowers grid stress, and adds to overall grid support.
In addition to its role of aggregating and optimizing DERs, a VPP offers a range of essential ancillary services. These services include frequency regulation. This is achieved by maintaining grid frequency within acceptable bounds through rapid power adjustment [ 136 , 137 , 138 , 139 ]. VPPs also provide voltage support by injecting or absorbing reactive power to stabilize voltage levels [ 80 , 140 , 141 ].
Moreover, VPPs contribute to peak regulation, managing demand during high load periods to alleviate grid stress [ 142 , 143 , 144 ]. The comprehensive suite of ancillary services offered by VPPs ensures grid stability, enhances reliability, and facilitates the integration of RESs, making them vital assets in modern power systems.
Demand response and load management: A VPP inherent components of demand response and load control enable effective energy usage. By actively communicating with connected consumers to alter electricity consumption in response to grid circumstances and price signals, VPPs participate in demand response. In order to avoid peak demand times and lessen grid load, VPPs optimize the scheduling of operations and equipment that consume a lot of electricity [ 59 , 81 , 96 ]. This demand-side flexibility not only supports grid stability, but also empowers consumers to actively participate in energy conservation, contributing to a sustainable energy ecosystem [ 66 , 145 ]. The VPP’s ability to efficiently balance energy supply and demand through demand response and load management strategies makes it a pivotal stakeholder in modern power systems.
The technical aspects of VPPs represent a dynamic and transformative force in the energy sector. VPPs provide effective renewable energy integration, grid stability, and demand response capabilities by aggregating and optimizing various DERs.
Market/economical aspect of VPP
VPPs provide an appealing scenario for the future of energy systems in terms of their commercial and financial prepositions. VPPs can completely alter the economics of electricity generation and consumption as they are dynamic aggregators of various DERs. VPPs maximize the use of DERs, optimize income generation, and improve participation in the energy market [ 11 ]. The VPP does this via real-time data analytics, complex forecasting algorithms, and clever energy trading methods. As a result of their capacity to offer a versatile and dispatchable portfolio of assets (DERs), VPPs are better equipped to meet swiftly to dynamic market conditions, such as energy pricing and demand patterns. VPPs deliver a strong economic case for sustainability, affordability, and resilience in the energy ecosystem by making it possible to efficiently deploy renewable sources of energy, support demand response programs, and provide ancillary services to the grid. VPPs technology’s commercial implications hold significant promise for developing a more effective, competitive, and customer-focused energy landscape as it continues to advance.
Currently, the majority of jurisdictions have already started deregulation or liberalization and competition-opening process in their individual power markets [ 11 ]. In order to finance new infrastructure investments, increase the economic efficiency of power company operations, and particularly lower the ultimate prices of electricity delivery, deregulation or privatization has been advocated [ 146 ]. A vertical structure as stipulated by [ 146 ], where all activities were merged, was replaced with an organization where generation, transmission, distribution, and commerce work separately as a result of this reform in the energy sector.
Additionally, the large integration of renewables into the power grid that characterizes the contemporary energy landscape suggests a greater need for the system’s balancing mechanism due to the random nature of the RESs generation schedule. One significant benefit of VPPs is that they boost their shared profit by selling energy on behalf of the DER owners to improve the balancing mechanism when they access the wholesale electricity markets. The participation of VPPs in various electricity markets is covered in this section.
Day-ahead market: Day-ahead market refers to the buying and selling of electricity on the day before the actual production and delivery. VPPs actively participate in the day-ahead market by supplying their aggregated portfolio of DERs for electricity trading. VPPs forecast energy generation trends for the next day using advanced forecasting and data analytics. Based on these insights and market prices, VPPs strategically bid these aggregated resources to optimize revenue generation [ 84 , 147 , 148 , 149 , 150 , 151 ].
Ancillary service market: VPPs actively participate in the ancillary services market by providing critical assistance to the electric grid. The VPP does this by dynamically altering the output of their aggregated DERs. VPPs respond in real-time to grid signals to maintain stability, assure a continuous power supply, and improve grid reliability. With this, VPPs play an important role in supporting grid operations and optimizing grid performance. Several studies have incorporated the ability to engage in ancillary services markets into VPP modeling in order to enable regulation that ensures the security of electricity supply [ 26 , 143 , 150 , 152 , 153 , 154 , 155 , 156 ].
Reserve market: In the reserve market, VPPs actively participate by offering their combined output of DER as a reserve capacity to support the grid’s reliability. VPPs reserve a portion of their generated power from the DERs, ready to be dispatched within short notice to address sudden changes in electricity demand and supply or even an outage of grid operator’s outage of generators. By participating in the reserve market, VPPs offer a valuable and flexible solution for grid operators to maintain grid reliability. As VPP technology advances, their involvement in the reserve market will become ever more vital in contributing to the efficient and secure operation of the electric grid. Various strategies to make ideal or optimal reserve market decisions have been studied in several papers. According to the findings of these studies, the reserve market is more significant at times of peak demand since a contingency can have a higher impact [ 26 , 127 , 157 , 158 , 159 , 160 ].
Intra-day/real-time market: The VPP actively participates in the intra-day market by precisely adjusting the energy traded in the day-ahead market. The VPP strategically optimizes its DER dispatch and offers flexible resources in response to dynamic market prices and grid needs [ 11 ].
Although intraday markets enable VPPs to adjust scheduled energy after the day-ahead market, an exchange power imbalance may still emerge as the dispatch time approaches. VPPs can thus participate in real-time balancing markets to avoid penalties. The goal of the real-time market is to reduce the imbalance errors and their associated cost. The various electricity markets in which the VPP participates are provided in Table 5 to outline the key characteristics. Figure 4 also gives a graphical analysis of the key characteristics of the electricity market that the VPP operates in.
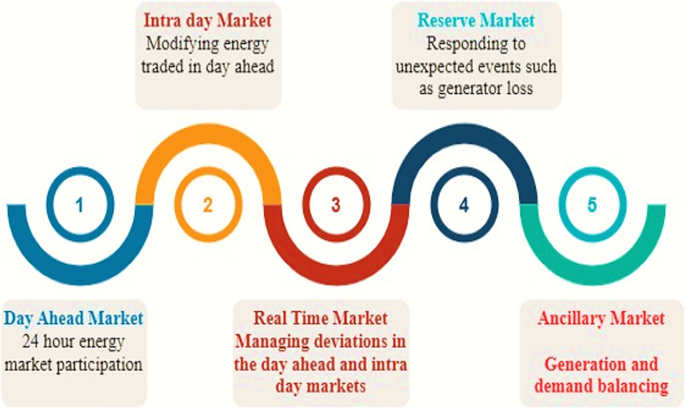
Electricity markets characteristics
Real-world implementation of VPPs
VPPs in the real world provide fascinating insights on their revolutionary impact on contemporary power systems. VPP implementations around the world demonstrate their adaptability in maximizing DERs. These examples elaborate on the value of VPPs in grid stability, renewable generation, and demand response. VPP projects are becoming more common, proving their potential to revolutionize energy systems. The VPP market is expected to grow from $1.3billion in 2019 to $5.9billion in 2027, with a compound annual growth rate of 21.3% from 2020 to 2027 [ 25 ]. In Norway, Statkraf is the world’s largest VPP with a capacity of 10GW from over 1000 aggregated assets. Recently, Tesla announced to scale up the south Australia VPP which connects assets from 4000 to 50,000 homes, which will make it the world’s largest VPP [ 172 ]. Storing and distributing power from residential and commercial customers, Tesla’s Powerpacks and Powerwall promote grid dependability and the integration of renewable energy. These real-world examples demonstrate how important VPPs are in creating a global energy ecosystem that is robust, efficient, and sustainable. Selected real-world applications [ 124 , 172 ] are summarized in Table 6 .
Applications of VPPs in the real world have offered an important lesson that will guide their development, deployment, and scalability. Key insights from these applications include the following but not limited to:
Flexibility and scalability: The significance of developing flexible and scalable systems has been shown by the successful VPP deployments. VPPs support a variety of DERs and adjust to shifting market dynamics and grid conditions.
Integration of DERs: For the VPP to operate at its best, several DERs must be integrated into a single, coordinated system. Advanced data analytics and control algorithms are essential for managing DERs efficiently and maximizing their contributions, as demonstrated by real-world applications.
Interoperability and interconnection: VPPs generally operate in sophisticated energy ecosystems with a variety of stakeholders. Smooth VPP integration and operation require interoperability and seamless interconnection with grid operators, and other market participants.
Market participation: The significance of active market participation has been emphasized by real-world VPP applications. Using effective energy trading techniques and intelligent bidding in electricity markets. VPPs can maximize income production and assist the integration of RESs at a fair price.
The ongoing development and deployment of VPPs can be improved by taking lessons from these practical applications, ensuring that they continue to contribute to a sustainable, effective, and decentralized energy future.
However, despite the successes chalked up by these projects, there are still challenges that must be addressed. Cybersecurity threats, consumer engagement, data management and analytics, achieving a positive return on investment and profitability are some of the model challenges that these projects face. Collaboration between stakeholders is necessary to overcome these obstacles.
Conclusions
VPPs have become transformative solutions revolutionizing the modern energy landscape. Applications in the real world have sounded their importance and have also demonstrated the adaptability and advantages of VPPs. VPPs have shown that they can promote the integration of renewable energy sources, aggregate and optimize a variety of DERs, and facilitate effective demand response.
Flexibility and scalability, which enable seamless adaptability to shifting grid conditions and market dynamics, have been shown to be essential for successful VPP adoption. VPPs have been able to improve cost-effective renewable energy integration and optimize revenue generation through active market participation and smart bidding tactics. Additionally, for VPPs including residential or commercial participants, consumer engagement and education are crucial for assuring buy-in and demand response programs.
Embracing the lessons learnt in the referenced literature, a VPP stands as a pivotal enabler in our journey towards a sustainable, decentralized, and resilient energy future. There can be an effective and customer-focused energy ecosystem that leads the path for a greener and more sustainable society by fully utilizing VPPs and maximizing their important contributions.
The ability of VPPs to maximize DERs, boost renewable energy integration, and improve grid stability makes them a crucial element in reaching a sustainable energy future. A VPP has the undisputed potential to change the energy landscape. The successful operation of VPPs in the modern era depends on a judicious blend of cutting-edge technology, supportive regulatory frameworks, and seamless connectivity with the existing electricity infrastructure. The aggregation and control of various DERs can be optimized by using real-time data analytics, artificial intelligence, and smart grid technologies. However, VPPs must overcome several obstacles, such as data security, grid interconnection, and scalability to realize their full potential. In a dynamic energy environment, taking care of these issues is essential to ensure the proper operation of VPPs.
Also, the development of flexible regulatory frameworks that support VPP implementation and market involvement is essential for the efficient operation of VPPs. The seamless integration of VPPs into current energy markets and the promotion of novel business models are made possible by clear regulations on market access, price structures, and grid services. Overall, an effective operation of VPPs in this era and beyond will depend on the following:
Advanced technological integration such as data analytics, smart grid technologies which are vital real-time data processing, accurate forecasting, and efficient optimization.
Regulatory support to encourage supportive and accommodative regulatory frameworks that will promote VPP deployment, and market participation.
Implementation of robust data security measures to protect sensitive information, guarantee consumer privacy, and safeguard against potential cyberattacks.
Implementing these recommendations will help shape and harness the potential of VPPs to transform the energy industry. With correct planning, VPPs will significantly contribute to the modern era’s goals of energy resource optimization, grid stability enhancement, and improved integration of RESs.
Availability of data and materials
Data sharing is not applicable to this article as no datasets were generated or analyzed during this work.
Abbreviations
Artificial Neural Network
Biogas/biomass power
Battery energy storage system
Combined heat and power
Convolutional Neural Network
Controlled load
- Distributed energy resources
Distributed generation
Distribution system operator
Energy storage system
European Union
Electric vehicles
Gas turbine
Heat pump power
Heating, ventilation, and air conditioning
Internet of Things
Long short-term memory
Mixed Integer Linear Programming
Model predictive control
Nuclear power
Pumped hydro storage
Programmable logic control
Power System Network
Particle Swarm Optimization
Photovoltaic
Renewable energy resources
- Renewable energy sources
Sustainable Development Goals
Thermal power
Transmission system operator
United Nations
Virtual power plant
Wind turbine
Zhao J, Patwary AK, Qayyum A et al (2022) The determinants of renewable energy sources for the fueling of green and sustainable economy. Energy 238:122029. https://doi.org/10.1016/j.energy.2021.122029
Article Google Scholar
Rapier R Global Energy Trends: insights from the 2023 Statistical Review of World Energy. In: Forbes. https://www.forbes.com/sites/rrapier/2023/08/06/global-energy-trends-insights-from-the-2023-statistical-review-of-world-energy/ . Accessed 12 Oct 2023
Liu J, Hu H, Yu SS, Trinh H (2023) Virtual power plant with renewable energy sources and energy storage systems for sustainable power grid-formation. Control Tech Demand Response Energies 16:3705. https://doi.org/10.3390/en16093705
Electricity demand globally 2040. In: Statista. https://www.statista.com/statistics/1118777/electricity-demand-worldwide/ . Accessed 2 Oct 2023
The Impact of Distributed Generation on Power Distribution. In: Util. One. https://utilitiesone.com/the-impact-of-distributed-generation-on-power-distribution . Accessed 26 Feb 2024
Strezoski L, Padullaparti H, Ding F, Baggu M (2022) Integration of utility distributed energy resource management system and aggregators for evolving distribution system operators. J Mod Power Syst Clean Energy 10:277–285. https://doi.org/10.35833/MPCE.2021.000667
Abedrabboh K, Karaki A, Al-Fagih L (2023) A combinatorial double auction for community shared distributed energy resources. IEEE Access 11:28355–28369. https://doi.org/10.1109/ACCESS.2023.3260022
Poudel S, Keene SJ, Kini RL et al (2022) Modeling environment for testing a distributed energy resource management system (DERMS) using GridAPPS-D platform. IEEE Access 10:77383–77395. https://doi.org/10.1109/ACCESS.2022.3192845
Tan Z, Zhong H, Xia Q et al (2020) Estimating the robust P-Q capability of a technical virtual power plant under uncertainties. IEEE Trans Power Syst 35:4285–4296. https://doi.org/10.1109/TPWRS.2020.2988069
Babatunde OM, Munda JL, Hamam Y (2020) Power system flexibility: a review. Energy Rep 6:101–106. https://doi.org/10.1016/j.egyr.2019.11.048
Naval N, Yusta JM (2021) Virtual power plant models and electricity markets—a review. Renew Sustain Energy Rev 149:111393. https://doi.org/10.1016/j.rser.2021.111393
Khan R, Islam N, Das SK et al (2021) Energy sustainability-survey on technology and control of microgrid, smart grid and virtual power plant. IEEE Access 9:104663–104694. https://doi.org/10.1109/ACCESS.2021.3099941
Sarmiento-Vintimilla JC, Torres E, Larruskain DM, Pérez-Molina MJ (2022) Applications, operational architectures and development of virtual power plants as a strategy to facilitate the integration of distributed energy resources. Energies 15:775. https://doi.org/10.3390/en15030775
Johansson P, Vendel M, Nuur C (2020) Integrating distributed energy resources in electricity distribution systems: an explorative study of challenges facing DSOs in Sweden. Util Policy 67:101117. https://doi.org/10.1016/j.jup.2020.101117
Lopes JAP, Hatziargyriou N, Mutale J et al (2007) Integrating distributed generation into electric power systems: a review of drivers, challenges, and opportunities. Electr Power Syst Res 77:1189–1203. https://doi.org/10.1016/j.epsr.2006.08.016
Nadeem F, Aftab MA, Hussain SMS et al (2019) Virtual power plant management in smart grids with XMPP based IEC 61850 communication. Energies 12:2398. https://doi.org/10.3390/en12122398
Marinescu B, Gomis-Bellmunt O, Dorfler F et al (2022) Dynamic virtual power plant: a new concept for grid integration of renewable energy sources. IEEE Access 10:104980–104995. https://doi.org/10.1109/ACCESS.2022.3205731
Gough M, Santos SF, Lotfi M et al (2022) Operation of a technical virtual power plant considering diverse distributed energy resources. IEEE Trans Ind Appl 58:2547–2558. https://doi.org/10.1109/TIA.2022.3143479
Palensky P, Dietrich D (2011) Demand side management: demand response, intelligent energy systems, and smart loads. IEEE Trans Ind Inform 7:381–388. https://doi.org/10.1109/TII.2011.2158841
Pasetti M, Rinaldi S, Manerba D (2018) A virtual power plant architecture for the demand-side management of smart prosumers. Appl Sci 8:432. https://doi.org/10.3390/app8030432
Lan G, Zhang Z, Guo M, et al (2022) Research on Virtual Power Plants Participating in Ancillary Service Market. In: 2022 2nd international conference on electrical engineering and control science (IC2ECS). P. 979–985
Nosratabadi SM, Hooshmand R-A, Gholipour E (2017) A comprehensive review on microgrid and virtual power plant concepts employed for distributed energy resources scheduling in power systems. Renew Sustain Energy Rev 67:341–363. https://doi.org/10.1016/j.rser.2016.09.025
Goia B, Cioara T, Anghel I (2022) Virtual power plant optimization in smart grids: a narrative review. Future Internet 14:128. https://doi.org/10.3390/fi14050128
Panda S, Mohanty S, Rout PK, Sahu BK (2022) A conceptual review on transformation of micro-grid to virtual power plant: issues, modeling, solutions, and future prospects. Int J Energy Res 46:7021–7054. https://doi.org/10.1002/er.7671
Zhang J (2022) The concept, project, and current status of virtual power plant: a review. J Phys Conf Ser 2152:012059. https://doi.org/10.1088/1742-6596/2152/1/012059
Wang H, Riaz S, Mancarella P (2020) Integrated techno-economic modeling, flexibility analysis, and business case assessment of an urban virtual power plant with multi-market co-optimization. Appl Energy 259:114142. https://doi.org/10.1016/j.apenergy.2019.114142
Yan P, Wang L, Yang S, et al (2022) Review on the development and application of virtual power plant under the background of dual carbon. In: 2nd international conference on mechanical, electronics, and electrical and automation control (METMS 2022). SPIE, pp 654–665
Rouzbahani HM, Karimipour H, Lei L (2021) A review on virtual power plant for energy management. Sustain Energy Technol Assess 47:101370. https://doi.org/10.1016/j.seta.2021.101370
Feng Y, Jia H, Wang X et al (2023) Review of operations for multi-energy coupled virtual power plants participating in electricity market. Energy Rep 9:992–999. https://doi.org/10.1016/j.egyr.2023.04.149
Wang L, Guo Z, Zhang Y, et al (2021) A review of virtual power plant: concepts and essential issues. In: 2021 IEEE sustainable power and energy conference (iSPEC). pp 655–660
Yang Z, Liu J, Baskaran S et al (2010) Enabling renewable energy—and the future grid—with advanced electricity storage. JOM 62:14–23. https://doi.org/10.1007/s11837-010-0129-0
Romero-Cadaval E, Francois B, Malinowski M, Zhong Q-C (2015) Grid-connected photovoltaic plants: an alternative energy source, replacing conventional sources. IEEE Ind Electron Mag 9:18–32. https://doi.org/10.1109/MIE.2014.2362211
Awerbuch S, Preston A (2012) The virtual utility: accounting, technology & competitive aspects of the emerging industry. Springer Science & Business Media
Google Scholar
Dielmann K, van der Velden A (2003) Virtual power plants (VPP)—a new perspective for energy generation? In: Proceedings of the 9th international scientific and practical conference of students, post-graduates modern techniques and technologies; 2003. MTT 2003. pp 18–20
Caldon R, Patria AR, Turri R (2004) Optimisation algorithm for a virtual power plant operation. In: 39th international universities power engineering conference, vol. 2; 2004. UPEC 2004. pp 1058–1062
Pudjianto D, Ramsay C, Strbac G (2007) Virtual power plant and system integration of distributed energy resources. IET Renew Power Gener 1:10–16. https://doi.org/10.1049/iet-rpg:20060023
Pudjianto D, Ramsay C, Strbac G (2008) Microgrids and virtual power plants: Concepts to support the integration of distributed energy resources. Proc Inst Mech Eng Part J Power Energy 222:731–741. https://doi.org/10.1243/09576509JPE556
Ruiz N, Iñ C, Oyarzabal J (2009) A direct load control model for virtual power plant management. IEEE Trans Power Syst 24:959–966. https://doi.org/10.1109/TPWRS.2009.2016607
Asmus P (2010) Microgrids, virtual power plants and our distributed energy future. Electr J 23:72–82. https://doi.org/10.1016/j.tej.2010.11.001
Mashhour E, Moghaddas-Tafreshi SM (2011) Bidding strategy of virtual power plant for participating in energy and spinning reserve markets—part II: numerical analysis. IEEE Trans Power Syst 26:957–964. https://doi.org/10.1109/TPWRS.2010.2070883
Bremer J, Sonnenschein M (2014) Parallel tempering for constrained many criteria optimization in dynamic virtual power plants. In: 2014 IEEE symposium on computational intelligence applications in smart grid (CIASG). pp 1–8
Al-Awami AT, Amleh NA, Muqbel AM (2017) Optimal demand response bidding and pricing mechanism with fuzzy optimization: application for a virtual power plant. IEEE Trans Ind Appl 53:5051–5061. https://doi.org/10.1109/TIA.2017.2723338
Koraki D, Strunz K (2018) Wind and solar power integration in electricity markets and distribution networks through service-centric virtual power plants. IEEE Trans Power Syst 33:473–485. https://doi.org/10.1109/TPWRS.2017.2710481
Yu S, Fang F, Liu Y, Liu J (2019) Uncertainties of virtual power plant: Problems and countermeasures. Appl Energy 239:454–470. https://doi.org/10.1016/j.apenergy.2019.01.224
Mahmud K, Khan B, Ravishankar J et al (2020) An internet of energy framework with distributed energy resources, prosumers, and small-scale virtual power plants: an overview. Renew Sustain Energy Rev 127:109840. https://doi.org/10.1016/j.rser.2020.109840
Peng D, Poudineh R (2019) Electricity market design under increasing renewable energy penetration: Misalignments observed in the European Union. Util Policy 61:100970. https://doi.org/10.1016/j.jup.2019.100970
Liu R, Liu Y, Jing Z (2020) Impact of industrial virtual power plant on renewable energy integration. In: 2020 IEEE/IAS industrial and commercial power system Asia (I&CPS Asia). pp 1198–1202
Jin X, Wang J, Shen X, et al (2018) An overview of virtual power plant development from the perspective of market participation. In: 2018 2nd IEEE conference on energy internet and energy system integration (EI2). pp 1–6
Wang Z, Yang P, Liu S et al (2017) Coordination and optimization strategy of VPP considering demand response and multi-energy coordination. Electr Power Constr 38:60–66
Othman M, Hegazy YG, Abdelaziz A (2015) A review of virtual power plant definitions, components, framework and optimization. Int Electr Eng J IEEJ 6:2010–2024
Tong Y, Meng Z, Qiu P et al (2023) The carbon trading operation optimization for virtual power plants of industrial parks considering wind power. J Phys Conf Ser 2474:012032. https://doi.org/10.1088/1742-6596/2474/1/012032
Venkatachary SK, Alagappan A, Andrews LJB (2021) Cybersecurity challenges in energy sector (virtual power plants)—can edge computing principles be applied to enhance security? Energy Inform 4:5. https://doi.org/10.1186/s42162-021-00139-7
Hongliang W, Benjie L, Daoxin P, Ling W (2021) Virtual power plant participates in the two-level decision-making optimization of internal purchase and sale of electricity and external multi-market. IEEE Access 9:133625–133640. https://doi.org/10.1109/ACCESS.2021.3112549
Wang S, Jia R, Shi X et al (2022) Research on capacity allocation optimization of commercial virtual power plant (CVPP). Energies 15:1303. https://doi.org/10.3390/en15041303
Domingo-Mondejar ID (2022) A review of the evolution and main roles of virtual power plants as key stakeholders in power systems. IEEE Access 10:47937–47964. https://doi.org/10.1109/ACCESS.2022.3171823
Luo J, Gao Y, Yang W et al (2018) Optimal operation modes of virtual power plants based on typical scenarios considering output evaluation criteria. Energies 11:2634. https://doi.org/10.3390/en11102634
Zhang Y, Pan W, Lou X, et al (2021) Operation characteristics of virtual power plant and function design of operation management platform under emerging power system. In: 2021 international conference on power system technology (POWERCON). pp 194–196
Fan S, Xiao J, Li Z, He G (2022) Characterization and trading of energy level and energy shift considering virtual power plant. J Mod Power Syst Clean Energy 10:1784–1789. https://doi.org/10.35833/MPCE.2021.000192
Chantzis G, Papadopoulos AM, Giama E, Nizetic S (2023) The potential of demand response as a tool for decarbonization in the energy transition. Energy Build. https://doi.org/10.1016/j.enbuild.2023.113255
Behi B, Baniasadi A, Arefi A et al (2020) Cost-benefit analysis of a virtual power plant including solar PV, flow battery, heat pump, and demand management: a western Australian case study. Energies 13:2614. https://doi.org/10.3390/en13102614
Behi B, Arefi A, Jennings P et al (2021) Advanced monitoring and control system for virtual power plants for enabling customer engagement and market participation. Energies 14:1113. https://doi.org/10.3390/en14041113
Shen J, Jiang C, Li B (2015) Controllable load management approaches in smart grids. Energies 8:11187–11202. https://doi.org/10.3390/en81011187
Abbasi MH, Taki M, Rajabi A et al (2019) Coordinated operation of electric vehicle charging and wind power generation as a virtual power plant: a multi-stage risk constrained approach. Appl Energy 239:1294–1307. https://doi.org/10.1016/j.apenergy.2019.01.238
Hatziargyriou ND, Asimakopoulou GE (2020) DER integration through a monopoly DER aggregator. Energy Policy 137:111124. https://doi.org/10.1016/j.enpol.2019.111124
Tascikaraoglu A, Erdinc O, Uzunoglu M, Karakas A (2014) An adaptive load dispatching and forecasting strategy for a virtual power plant including renewable energy conversion units. Appl Energy 119:445–453. https://doi.org/10.1016/j.apenergy.2014.01.020
Oladimeji O, Ortega Á, Sigrist L, et al (2022) Modeling demand flexibility of res-based virtual power plants. In: 2022 IEEE power & energy society general meeting (PESGM). pp 1–5
Chen J, Liu M, Milano F (2021) Aggregated model of virtual power plants for transient frequency and voltage stability analysis. IEEE Trans Power Syst 36:4366–4375. https://doi.org/10.1109/TPWRS.2021.3063280
Prabatha T, Hager J, Carneiro B et al (2020) Analyzing energy options for small-scale off-grid communities: a Canadian case study. J Clean Prod 249:119320. https://doi.org/10.1016/j.jclepro.2019.119320
Lima MA, Mendes LFR, Mothé GA et al (2020) Renewable energy in reducing greenhouse gas emissions: reaching the goals of the Paris agreement in Brazil. Environ Dev 33:100504. https://doi.org/10.1016/j.envdev.2020.100504
Akaev AA, Davydova OI (2020) The Paris agreement on climate is coming into force: will the great energy transition take place? Her Russ Acad Sci 90:588–599. https://doi.org/10.1134/S1019331620050111
Muruganantham B, Gnanadass R, Padhy NP (2017) Challenges with renewable energy sources and storage in practical distribution systems. Renew Sustain Energy Rev 73:125–134. https://doi.org/10.1016/j.rser.2017.01.089
Goud BS, Reddy CR, Kalyan CN et al (2021) Grid integration of renewable energy sources using GA technique for improving power quality. Int J Renew Energy Res IJRER 11:1390–1402
Nekrasov SA (2021) Reducing costs for integration of renewable energy sources: a way to making renewable energy more accessible. Therm Eng 68:593–603. https://doi.org/10.1134/S0040601521070077
Bouali E-T, Abid MR, Boufounas E-M et al (2022) Renewable energy integration into cloud & IoT-based smart agriculture. IEEE Access 10:1175–1191. https://doi.org/10.1109/ACCESS.2021.3138160
Panda S, Dhaka RK, Panda B, et al (2022) A review on application of machine learning in solar energy & photovoltaic generation prediction. In: 2022 international conference on electronics and renewable systems (ICEARS). pp 1180–1184
Prema V, Bhaskar MS, Almakhles D et al (2022) Critical review of data, models and performance metrics for wind and solar power forecast. IEEE Access 10:667–688. https://doi.org/10.1109/ACCESS.2021.3137419
Wang H, Lei Z, Zhang X et al (2019) A review of deep learning for renewable energy forecasting. Energy Convers Manag 198:111799. https://doi.org/10.1016/j.enconman.2019.111799
Suo S, Kuang X, Cheng R, et al (2022) Research of real-time monitoring and control technology for distributed energy storage based on 5G. In: 2022 IEEE/IAS industrial and commercial power system Asia (I&CPS Asia). pp 1496–1500
Pal P, Parvathy AK, Devabalaji KR et al (2021) IoT-based real time energy management of virtual power plant using PLC for transactive energy framework. IEEE Access 9:97643–97660. https://doi.org/10.1109/ACCESS.2021.3093111
Moutis P, Georgilakis PS, Hatziargyriou ND (2018) Voltage regulation support along a distribution line by a virtual power plant based on a center of mass load modeling. IEEE Trans Smart Grid 9:3029–3038. https://doi.org/10.1109/TSG.2016.2624633
Hu D, Liu H, Zhu Y et al (2023) Demand response-oriented virtual power plant evaluation based on AdaBoost and BP neural network. Energy Rep 9:922–931. https://doi.org/10.1016/j.egyr.2023.05.012
Löschenbrand M (2021) A temporal neural network model for probabilistic multi-period forecasting of distributed energy resources. IEEE Access 9:147029–147041. https://doi.org/10.1109/ACCESS.2021.3121988
Amparore EG, Cinus F, Maestri C, et al (2021) Forecast of distributed energy generation and consumption in a partially observable electrical grid: a machine learning approach. In: 2021 IEEE Madrid PowerTech. pp 1–6
Vardhan BVS, Khedkar M, Srivastava I (2021) Cost effective day-ahead scheduling with stochastic load and intermittency forecasting for distribution system considering distributed energy resources. Energy Sour Part Recov Util Environ Eff. https://doi.org/10.1080/15567036.2021.1983669
Tripathy DS, Prusty BR (2021) Chapter 10—forecasting of renewable generation for applications in smart grid power systems. In: Tomar A, Kandari R (eds) Advances in smart grid power system. Academic Press, pp 265–298
Chapter Google Scholar
Zhou B, Meng Y, Huang W et al (2021) multi-energy net load forecasting for integrated local energy systems with heterogeneous prosumers. Int J Electr Power Energy Syst 126:106542. https://doi.org/10.1016/j.ijepes.2020.106542
Akhtar I, Kirmani S, Ahmad M, Ahmad S (2021) Average monthly wind power forecasting using fuzzy approach. IEEE Access 9:30426–30440. https://doi.org/10.1109/ACCESS.2021.3056562
Inteha A, Nahid-Al-Masood HF, Khan IA (2022) A data driven approach for day ahead short-term load forecasting. IEEE Access 10:84227–84243. https://doi.org/10.1109/ACCESS.2022.3197609
Abbasipour M, Igder MA, Liang X (2021) A novel hybrid neural network-based day-ahead wind speed forecasting technique. IEEE Access 9:151142–151154. https://doi.org/10.1109/ACCESS.2021.3126747
Nespoli A, Leva S, Mussetta M, Ogliari EGC (2022) A selective ensemble approach for accuracy improvement and computational load reduction in ANN-based PV power forecasting. IEEE Access 10:32900–32911. https://doi.org/10.1109/ACCESS.2022.3158364
Zheng J, Du J, Wang B et al (2023) A hybrid framework for forecasting power generation of multiple renewable energy sources. Renew Sustain Energy Rev 172:113046. https://doi.org/10.1016/j.rser.2022.113046
Maanavi M, Najafi A, Godina R et al (2019) Energy management of virtual power plant considering distributed generation sizing and pricing. Appl Sci 9:2817. https://doi.org/10.3390/app9142817
Raab AF, Ferdowsi M, Karfopoulos E, et al (2011) Virtual power plant control concepts with electric vehicles. In: 2011 16th international conference on intelligent system applications to power systems. pp 1–6
Béguin A, Nicolet C, Kawkabani B, Avellan F (2014) Virtual power plant with pumped storage power plant for renewable energy integration. In: 2014 international conference on electrical machines (ICEM). pp 1736–1742
Zafred K, Nieto-Martin J, Butans E (2016) Electric vehicles—effects on domestic low voltage networks. In: 2016 IEEE international energy conference (ENERGYCON). pp 1–6
Liu Z, Zheng W, Qi F et al (2018) Optimal dispatch of a virtual power plant considering demand response and carbon trading. Energies 11:1488. https://doi.org/10.3390/en11061488
Shropshire D, Purvins A, Papaioannou I, Maschio I (2012) Benefits and cost implications from integrating small flexible nuclear reactors with offshore wind farms in a virtual power plant. Energy Policy 46:558–573. https://doi.org/10.1016/j.enpol.2012.04.037
Rappaport RD, Miles J (2017) Cloud energy storage for grid scale applications in the UK. Energy Policy 109:609–622. https://doi.org/10.1016/j.enpol.2017.07.044
Mazzi N, Trivella A, Morales JM (2019) Enabling active/passive electricity trading in dual-price balancing markets. IEEE Trans Power Syst 34:1980–1990. https://doi.org/10.1109/TPWRS.2018.2888937
Sun G, Qian W, Huang W et al (2019) Stochastic adaptive robust dispatch for virtual power plants using the binding scenario identification approach. Energies 12:1918. https://doi.org/10.3390/en12101918
Liu J, Li J, Xiang Y et al (2019) Optimal sizing of cascade hydropower and distributed photovoltaic included virtual power plant considering investments and complementary benefits in electricity markets. Energies 12:952. https://doi.org/10.3390/en12050952
Pandžić H, Morales JM, Conejo AJ, Kuzle I (2013) Offering model for a virtual power plant based on stochastic programming. Appl Energy 105:282–292. https://doi.org/10.1016/j.apenergy.2012.12.077
Handschin E, Neise F, Neumann H, Schultz R (2006) Optimal operation of dispersed generation under uncertainty using mathematical programming. Int J Electr Power Energy Syst 28:618–626. https://doi.org/10.1016/j.ijepes.2006.03.003
Schulz C, Roder G, Kurrat M (2005) Virtual power plants with combined heat and power micro-units. In: 2005 international conference on future power systems. pp 5
Hany Elgamal A, Kocher-Oberlehner G, Robu V, Andoni M (2019) Optimization of a multiple-scale renewable energy-based virtual power plant in the UK. Appl Energy 256:113973. https://doi.org/10.1016/j.apenergy.2019.113973
Zhang J, Xu Z, Xu W et al (2019) Bi-objective dispatch of multi-energy virtual power plant: deep-learning-based prediction and particle swarm optimization. Appl Sci 9:292. https://doi.org/10.3390/app9020292
Fang F, Yu S, Liu M (2020) An improved Shapley value-based profit allocation method for CHP-VPP. Energy 213:118805. https://doi.org/10.1016/j.energy.2020.118805
Liu C, Yang RJ, Yu X et al (2021) Virtual power plants for a sustainable urban future. Sustain Cities Soc 65:102640. https://doi.org/10.1016/j.scs.2020.102640
Collath N, Tepe B, Englberger S et al (2022) Aging aware operation of lithium-ion battery energy storage systems: a review. J Energy Storage 55:105634. https://doi.org/10.1016/j.est.2022.105634
Stroe DI (2014) Lifetime models for lithium-ion batteries used in virtual power plant applications. Aalborg University, Department of Energy Technology
Behi B, Arefi A, Jennings P, et al (2020) Consumer engagement in virtual power plants through gamification. In: 2020 5th international conference on power and renewable energy (ICPRE). pp 131–137
Zurborg A (2010) Unlocking customer value: the virtual power plant. PowerWorld. https://www.energy.gov/oe/articles/unlocking-customer-value-virtual-power-plant
Henderson K, Loreau M (2023) A model of sustainable development goals: challenges and opportunities in promoting human well-being and environmental sustainability. Ecol Model 475:110164. https://doi.org/10.1016/j.ecolmodel.2022.110164
Onu Fergus U, Akpan Abasiam G (2017) Leveraging ICT for power delivery and electrification in Africa: cyber security, privacy and data protection. Int J Res 4:912–915
Sharghivand N, Derakhshan F (2021) Data security and privacy in industrial IoT. In: Karimipour H, Derakhshan F (eds) AI-enabled threat detection and security analysis for industrial IoT. Springer International Publishing, Cham, pp 21–39
Yang Q, Wang H, Wang T et al (2021) Blockchain-based decentralized energy management platform for residential distributed energy resources in a virtual power plant. Appl Energy 294:117026. https://doi.org/10.1016/j.apenergy.2021.117026
Maglaras LA, Ferrag MA, Janicke H et al (2022) Reliability, security, and privacy in power grids. Computer 55:85–88. https://doi.org/10.1109/MC.2022.3184425
Tufail S, Parvez I, Batool S, Sarwat A (2021) A survey on cybersecurity challenges, detection, and mitigation techniques for the smart grid. Energies 14:5894. https://doi.org/10.3390/en14185894
Alfiah F, Prastiwi NR (2022) Cyber security in smart grid technology: a systematic review. Int J Cyber IT Serv Manag 2:48–54
Khare U, Malviya A, Kumar Gawre S, Arya A (2023) Cyber physical security of a smart grid: a review. In: 2023 IEEE international students’ conference on electrical, electronics and computer science (SCEECS). pp 1–6
Shi J, Ma L, Li C et al (2022) A comprehensive review of standards for distributed energy resource grid-integration and microgrid. Renew Sustain Energy Rev 170:112957. https://doi.org/10.1016/j.rser.2022.112957
Saqib N, Duran IA, Sharif I (2022) Influence of energy structure, environmental regulations, and human capital on ecological sustainability in EKC framework; evidence from MINT countries. Front Environ Sci 10:968405
Patel S, Parkins JR (2023) Assessing motivations and barriers to renewable energy development: insights from a survey of municipal decision-makers in Alberta, Canada. Energy Rep 9:5788–5798. https://doi.org/10.1016/j.egyr.2023.05.027
Sikorski T, Jasiński M, Ropuszyńska-Surma E et al (2020) A case study on distributed energy resources and energy-storage systems in a virtual power plant concept: technical aspects. Energies 13:3086. https://doi.org/10.3390/en13123086
Lin J, Zhang S, Yang B, et al (2021) Customer-side energy management considering the availability of renewable virtual power plants. In: Retracted on September 15, 2021the sixth international conference on information management and technology. association for computing machinery, New York, NY, USA, pp 1–5
Liu X (2022) Research on optimal dispatch method of virtual power plant considering various energy complementary and energy low carbonization. Int J Electr Power Energy Syst 136:107670. https://doi.org/10.1016/j.ijepes.2021.107670
Iraklis C, Smend J, Almarzooqi A, Ghaoud T (2021) Optimal scheduling of synthetic reserves provided by virtual power plants. In: 2021 international conference on electrical, computer, communications and mechatronics engineering (ICECCME). pp 1–7
Rahimi M, Ardakani FJ, Ardakani AJ (2021) Optimal stochastic scheduling of electrical and thermal renewable and non-renewable resources in virtual power plant. Int J Electr Power Energy Syst 127:106658. https://doi.org/10.1016/j.ijepes.2020.106658
Dunnan L, Yuan G, Weiye W, Jiahao L (2021) Optimal scheduling method of virtual power plant based on bi level programming. IOP Conf Ser Earth Environ Sci 687:012141. https://doi.org/10.1088/1755-1315/687/1/012141
Zhang Y, Liu F, Wang Z et al (2022) Robust scheduling of virtual power plant under exogenous and endogenous uncertainties. IEEE Trans Power Syst 37:1311–1325. https://doi.org/10.1109/TPWRS.2021.3105418
Abdolrasol GMM, Hannan MA, Hussain SMS et al (2021) Energy management scheduling for microgrids in the virtual power plant system using artificial neural networks. Energies 14:6507. https://doi.org/10.3390/en14206507
Heydarian-Forushani E, Elghali SB, Zerrougui M, et al (2021) A centralized-stochastic solution for smart energy management in a virtual power plant. In: 2021 IEEE international conference on environment and electrical engineering and 2021 IEEE industrial and commercial power systems Europe (EEEIC/I&CPS Europe). pp 1–5
Ul Ali Binte Wasif J, Kazmi SAA, Altamimi A et al (2022) Smart energy management in virtual power plant paradigm with a new improved multilevel optimization based approach. IEEE Access 10:50062–50077. https://doi.org/10.1109/ACCESS.2022.3169707
Yin S, Ai Q, Li Z et al (2020) Energy management for aggregate prosumers in a virtual power plant: a robust Stackelberg game approach. Int J Electr Power Energy Syst 117:105605. https://doi.org/10.1016/j.ijepes.2019.105605
Oshnoei A, Kheradmandi M, Blaabjerg F et al (2022) Coordinated control scheme for provision of frequency regulation service by virtual power plants. Appl Energy 325:119734. https://doi.org/10.1016/j.apenergy.2022.119734
Hu Q, Han R, Quan X et al (2022) Grid-forming inverter enabled virtual power plants with inertia support capability. IEEE Trans Smart Grid 13:4134–4143. https://doi.org/10.1109/TSG.2022.3141414
Chen W, Qiu J, Zhao J et al (2021) Bargaining game-based profit allocation of virtual power plant in frequency regulation market considering battery cycle life. IEEE Trans Smart Grid 12:2913–2928. https://doi.org/10.1109/TSG.2021.3053000
Yang J, Zheng Q, Zhao J, et al (2017) Control strategy of virtual power plant participating in the system frequency regulation service. In: 2017 4th international conference on systems and informatics (ICSAI). pp 324–328
Jiazhen Wang, Xinwei Shen, Yinliang Xu, et al (2018) Ancillary service for frequency regulation based on multi-energy virtual power plant aggregating factory load. In: 11th IET international conference on advances in power system control, operation and management (APSCOM 2018). Institution of Engineering and Technology, Hong Kong, China, p 51 (7 pp.)-51 (7 pp.)
Zhong W, Tzounas G, Milano F (2022) Improving the power system dynamic response through a combined voltage-frequency control of distributed energy resources. IEEE Trans Power Syst 37:4375–4384. https://doi.org/10.1109/TPWRS.2022.3148243
Liu Q, Wang Y, Wang S et al (2022) Voltage regulation strategy for DC distribution networks based on coordination of centralized control and adaptive droop control. IEEE Trans Power Deliv 37:3730–3739. https://doi.org/10.1109/TPWRD.2021.3135884
Guili Y, Sixuan C, Xiaoxuan D (2021) Research on two-stage game strategy of virtual power plant in deep peak regulation auxiliary service market. E3S Web Conf 256:01026. https://doi.org/10.1051/e3sconf/202125601026
Li Y, Deng Y, Wang Y et al (2023) Robust bidding strategy for multi-energy virtual power plant in peak-regulation ancillary service market considering uncertainties. Int J Electr Power Energy Syst 151:109101. https://doi.org/10.1016/j.ijepes.2023.109101
Ya L, Deliang Z, Xuanyuan W (2019) A peak regulation ancillary service optimal dispatch method of virtual power plant based on reinforcement learning. In: 2019 IEEE innovative smart grid technologies—Asia (ISGT Asia). pp 4356–4361
Rashidizadeh-Kermani H, Vahedipour-Dahraie M, Shafie-khah M, et al (2020) Optimal scheduling of a virtual power plant with demand response in short-term electricity market. In: 2020 IEEE 20th Mediterranean electrotechnical conference (MELECON). pp 599–604
Liu H, Khan AR, Aslam S et al (2022) Financial impact of energy efficiency and energy policies aimed at power sector reforms: mediating role of financing in the power sector. Environ Sci Pollut Res 29:18891–18904. https://doi.org/10.1007/s11356-021-16882-z
Michael NE, Hasan S, Al-Durra A, Mishra M (2023) Economic scheduling of virtual power plant in day-ahead and real-time markets considering uncertainties in electrical parameters. Energy Rep 9:3837–3850. https://doi.org/10.1016/j.egyr.2023.02.092
Hadayeghparast S, SoltaniNejad Farsangi A, Shayanfar H (2019) Day-ahead stochastic multi-objective economic/emission operational scheduling of a large scale virtual power plant. Energy 172:630–646. https://doi.org/10.1016/j.energy.2019.01.143
Yang D, He S, Wang M, Pandžić H (2020) Bidding strategy for virtual power plant considering the large-scale integrations of electric vehicles. IEEE Trans Ind Appl 56:5890–5900. https://doi.org/10.1109/TIA.2020.2993532
Shayegan-Rad A, Badri A, Zangeneh A (2017) Day-ahead scheduling of virtual power plant in joint energy and regulation reserve markets under uncertainties. Energy 121:114–125. https://doi.org/10.1016/j.energy.2017.01.006
Dabhi D, Pandya K (2020) Metaheuristic optimization algorithm for day-ahead energy resource management (ERM) in microgrid environment of power system. In: Mehta A, Rawat A, Chauhan P (eds) Recent advances in communication infrastructure. Springer, Singapore, pp 115–125
Tajeddini MA, Rahimi-Kian A, Soroudi A (2014) Risk averse optimal operation of a virtual power plant using two stage stochastic programming. Energy 73:958–967. https://doi.org/10.1016/j.energy.2014.06.110
Zhou B, Liu X, Cao Y et al (2016) Optimal scheduling of virtual power plant with battery degradation cost. IET Gener Transm Distrib 10:712–725. https://doi.org/10.1049/iet-gtd.2015.0103
Heredia F-J, Cuadrado MD, Corchero C (2018) On optimal participation in the electricity markets of wind power plants with battery energy storage systems. Comput Oper Res 96:316–329. https://doi.org/10.1016/j.cor.2018.03.004
Fusco A, Gioffrè D, Francesco Castelli A et al (2023) A multi-stage stochastic programming model for the unit commitment of conventional and virtual power plants bidding in the day-ahead and ancillary services markets. Appl Energy 336:120739. https://doi.org/10.1016/j.apenergy.2023.120739
Jia H, Wang X, Zhang X, Liu D (2023) Optimal operation of virtual power plants participating in auxiliary service market coordinating with energy storage allocation. In: Jia H, Wang X, Zhang X, Liu D (eds) Business models and reliable operation of virtual power plants. Singapore, Springer Nature, pp 69–90
Baringo A, Baringo L, Arroyo JM (2019) Day-ahead self-scheduling of a virtual power plant in energy and reserve electricity markets under uncertainty. IEEE Trans Power Syst 34:1881–1894. https://doi.org/10.1109/TPWRS.2018.2883753
Alahyari A, Ehsan M, Mousavizadeh M (2019) A hybrid storage-wind virtual power plant (VPP) participation in the electricity markets: a self-scheduling optimization considering price, renewable generation, and electric vehicles uncertainties. J Energy Storage 25:100812. https://doi.org/10.1016/j.est.2019.100812
Ju L, Tan Q, Lu Y et al (2019) A CVaR-robust-based multi-objective optimization model and three-stage solution algorithm for a virtual power plant considering uncertainties and carbon emission allowances. Int J Electr Power Energy Syst 107:628–643. https://doi.org/10.1016/j.ijepes.2018.12.012
Zhou Y, Wei Z, Sun G et al (2019) Four-level robust model for a virtual power plant in energy and reserve markets. IET Gener Transm Distrib 13:2036–2043. https://doi.org/10.1049/iet-gtd.2018.5197
(2021) Optimal scheduling of synthetic reserves provided by virtual power plants. https://doi.org/10.1109/ICECCME52200.2021.9591118
Ziegler C, Richter A, Hauer I, Wolter M (2018) Technical integration of virtual power plants enhanced by energy storages into German system operation with regard to following the schedule in intra-day. In: 2018 53rd international universities power engineering conference (UPEC). pp 1–6
Wozabal D, Rameseder G (2020) Optimal bidding of a virtual power plant on the Spanish day-ahead and intraday market for electricity. Eur J Oper Res 280:639–655. https://doi.org/10.1016/j.ejor.2019.07.022
Article MathSciNet Google Scholar
Toubeau J-F, De Grève Z, Vallée F (2018) Medium-term multimarket optimization for virtual power plants: a stochastic-based decision environment. IEEE Trans Power Syst 33:1399–1410. https://doi.org/10.1109/TPWRS.2017.2718246
Ko R, Kang D, Joo S-K (2019) Mixed integer quadratic programming based scheduling methods for day-ahead bidding and intra-day operation of virtual power plant. Energies 12:1410. https://doi.org/10.3390/en12081410
Kong X, Xiao J, Wang C et al (2019) Bi-level multi-time scale scheduling method based on bidding for multi-operator virtual power plant. Appl Energy 249:178–189. https://doi.org/10.1016/j.apenergy.2019.04.130
Rahimiyan M, Baringo L (2019) Real-time energy management of a smart virtual power plant. IET Gener Transm Distrib 13:2015–2023. https://doi.org/10.1049/iet-gtd.2018.5637
Tang W, Yang H-T (2019) Optimal operation and bidding strategy of a virtual power plant integrated with energy storage systems and elasticity demand response. IEEE Access 7:79798–79809. https://doi.org/10.1109/ACCESS.2019.2922700
Gao R, Guo H, Zhang R et al (2019) A two-stage dispatch mechanism for virtual power plant utilizing the CVaR Theory in the electricity spot market. Energies 12:3402. https://doi.org/10.3390/en12173402
Hu J, Jiang C, Liu Y (2019) Short-term bidding strategy for a price-maker virtual power plant based on interval optimization. Energies 12:3662. https://doi.org/10.3390/en12193662
Wu H, Liu X, Ye B, Xu B (2020) Optimal dispatch and bidding strategy of a virtual power plant based on a Stackelberg game. IET Gener Transm Distrib 14:552–563. https://doi.org/10.1049/iet-gtd.2019.0493
Virtual Power Plants: The Future of Renewable Power? https://www.linkedin.com/pulse/virtual-power-plants-future-renewable-ashik-kalam . Accessed 19 Sept 2023
Download references
Acknowledgements
Authors express their sincere gratitude to Professor Farrag of School of Computing, Engineering and the Built Environment at Glasgow Caledonian University for proofreading and providing valuable insights in enhancing the accuracy, clarity, and readability of this manuscript.
Open access funding provided by The Science, Technology & Innovation Funding Authority (STDF) in cooperation with The Egyptian Knowledge Bank (EKB).
Author information
Authors and affiliations.
Department of Electrical Power Engineering, Egypt Japan University of Science and Technology, New Borg El-Arab City, Egypt
Sobhy Abdelkader, Jeremiah Amissah & Omar Abdel-Rahim
Electrical Engineering Department, Mansoura University, Mansoura, 35516, Egypt
Sobhy Abdelkader
Electrical Engineering Department, Aswan University, Aswan, Egypt
Omar Abdel-Rahim
You can also search for this author in PubMed Google Scholar
Contributions
SA set the main topic of the paper. JA searched for and collected most of the references. All authors contributed in analysis and writing. OA and SA worked on the review comments and carried out the required amendments. All the authors reviewed and approved the final version before submission.
Corresponding author
Correspondence to Sobhy Abdelkader .
Ethics declarations
Ethics approval and consent to participate.
Not applicable.
Consent for publication
Competing interests.
The authors declare no competing interests.
Additional information
Publisher's note.
Springer Nature remains neutral with regard to jurisdictional claims in published maps and institutional affiliations.
Rights and permissions
Open Access This article is licensed under a Creative Commons Attribution 4.0 International License, which permits use, sharing, adaptation, distribution and reproduction in any medium or format, as long as you give appropriate credit to the original author(s) and the source, provide a link to the Creative Commons licence, and indicate if changes were made. The images or other third party material in this article are included in the article's Creative Commons licence, unless indicated otherwise in a credit line to the material. If material is not included in the article's Creative Commons licence and your intended use is not permitted by statutory regulation or exceeds the permitted use, you will need to obtain permission directly from the copyright holder. To view a copy of this licence, visit http://creativecommons.org/licenses/by/4.0/ .
Reprints and permissions
About this article
Cite this article.
Abdelkader, S., Amissah, J. & Abdel-Rahim, O. Virtual power plants: an in-depth analysis of their advancements and importance as crucial players in modern power systems. Energ Sustain Soc 14 , 52 (2024). https://doi.org/10.1186/s13705-024-00483-y
Download citation
Received : 14 August 2023
Accepted : 07 August 2024
Published : 27 August 2024
DOI : https://doi.org/10.1186/s13705-024-00483-y
Share this article
Anyone you share the following link with will be able to read this content:
Sorry, a shareable link is not currently available for this article.
Provided by the Springer Nature SharedIt content-sharing initiative
- Contemporary power systems
- Virtual power plants
Energy, Sustainability and Society
ISSN: 2192-0567
- General enquiries: [email protected]
An IoT Enabled Energy Management System with Precise Forecasting and Load Optimization for PV Power Generation
- Original Article
- Published: 27 August 2024
Cite this article
- Challa Krishna Rao ORCID: orcid.org/0000-0003-3848-0778 1 , 2 ,
- Sarat Kumar Sahoo 1 &
- Franco Fernando Yanine 3
The challenge of demand-side energy management involves effectively leveraging renewable energy sources while mitigating power consumption constraints. The Intelligent Smart Energy Management System (ISEMS) aims to address this by accurately estimating energy availability and planning ahead for optimal usage. ISEMS utilizes Support Vector Machine (SVM) regression model with Particle Swarm Optimization (PSO) to forecast energy with high precision. Evaluation against other models demonstrates superior accuracy. Experimental setup of ISEMS is presented, showcasing its performance across different configurations, prioritizing user comfort. Additionally, integration of Internet of Things (IoT) enables user-end monitoring.
This is a preview of subscription content, log in via an institution to check access.
Access this article
Subscribe and save.
- Get 10 units per month
- Download Article/Chapter or eBook
- 1 Unit = 1 Article or 1 Chapter
- Cancel anytime
Price includes VAT (Russian Federation)
Instant access to the full article PDF.
Rent this article via DeepDyve
Institutional subscriptions
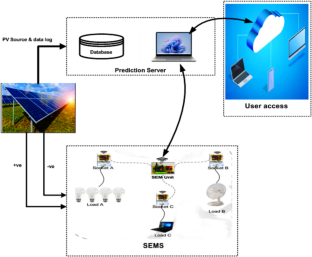
Explore related subjects
- Artificial Intelligence
Availability of Data and Materials
Generated Statement: The original contributions presented in the study are included in the article/supplementary material further inquiries can be directed to the corresponding author/s.
Abate F, Carratù M, Liguori C, Paciello V (2019) A low-cost smart power meter for IoT. Measurement 136:59–66
Article Google Scholar
Agyemang JO, Yu D, Kponyo J (2021) Autonomic IoT: towards smart system components with cognitive IoT. In: Proceedings of the pan-African artificial intelligence and smart systems conference, Windhoek, Namibia, 6–8 September 2021. Springer, Berlin
Ahmad T, Madonski R, Zhang D, Huang C, Mujeeb A (2022) Data-driven probabilistic machine learning in sustainable smart energy/smart energy systems: Key developments, challenges, and future research opportunities in the context of smart grid paradigm. Renew Sustain Energy Rev 160:112128
Ahmadi SE, Rezaei N, Khayyam H (2020) Energy management system of networked microgrids through optimal reliability-oriented day-ahead self-healing scheduling. Sustain Energy Grids Netw 23:100387
Alavi AH, Jiao P, Buttlar WG, Lajnef N (2018) Internet of things-enabled smart cities: state-of-the-art and future trends. Measurement 129(July):589–606
Aliyan E, Aghamohammadi M, Kia M, Heidari A, Shafie-khah M, Catalão JP (2020) Decision tree analysis to identify harmful contingencies and estimate blackout indices for predicting system vulnerability. Electr Power Syst Res 178:106036
Almaiah MA, Almomani O, Alsaaidah A, Al-Otaibi S, Bani-Hani N, Hwaitat AKA, Al-Zahrani A, Lutfi A, Awad AB, Aldhyani TH (2022) Performance investigation of principal component analysis for intrusion detection system using different support vector machine kernels. Electronics 11:3571
Asif M, Khan WU, Afzal HR, Nebhen J, Ullah I, Rehman AU, Kaabar MK (2021) Reduced-complexity LDPC decoding for next-generation IoT networks. Wirel Commun Mob Comput 2021:2029560
Bashar A, Rabbani MR, Khan S, Ali MAM (2021) Data-driven finance: Abibliometric review and scientific mapping. In: Proceedings of the 2021 International conference on data analytics for business and industry (ICDABI). pp 161–166
Bashir AK, Khan S, Prabadevi B, Deepa N, Alnumay WS, Gadekallu TR, Maddikunta PKR (2021) Comparative analysis of machine learning algorithms for predicting smart grid stability. Int Trans Electr Energy Syst 31:e12706
Beştepe F, Yildirim SÖ (2022) Acceptance of IoT-based and sustainability-oriented smart city services: a mixed methods study. Sustain Cities Soc 80:103794
Blasi S, Ganzaroli A, De Noni I (2022) Smartening sustainable development incities: strengthening the theoretical linkage between smart cities and SDGs. Sustain Cities Soc 80:103793
Dave B, Kubler S, Främling K, Koskela L (2020) Opportunities for enhanced lean construction management using Internet of Things standards. Int J Pervasive Comput Commun 61:86–97
Google Scholar
Demirezen G, Fung A, Deprez M (2020) Development and optimization of artificial neural network algorithms for the prediction of building specific local temperature for HVAC control. Int J Energy Res 44:8513–8531
Hamdani H, Pulungan AB, Myori DE, Elmubdi F, Hasannuddin T (2021) Real time monitoring system on solar panel orientation control using visual basic. J Appl Eng Technol Sci 2:112–124
Hossein Motlagh N, Mohammadrezaei M, Hunt J, Zakeri B (2020) Internet of things (IoT) and the energy sector. Energies 13(2):494
Huang Y, Wang L, Guo W, Kang Q, Wu Q (2016) Chance constrained optimization in a home energy management system. IEEE Trans Smart Grid 9(1):1
Khan R, Yang Q, Ullah I, Rehman AU, Tufail AB, Noor A, Rehman A, Cengiz K (2022) 3D convolutional neural networks based automatic modulation classification in the presence of channel noise. IET Commun 16:497–509
Krishna Rao C, Sahoo SK, Yanine FF (2023) An IoT-based intelligent smart energy monitoring system for solar PV power generation. In: Lublow M (ed) Energy harvesting and systems. Walter de Gruyter GmbH
Lekvan AA, Habibifar R, Moradi M, Khoshjahan M, Nojavan S, Jermsittiparsert K (2021) Robust optimization of renewable-basedmulti-energy micro-grid integrated with flexible energy conversion and storage devices. Sustain Cities Soc 64:102532
Lilis G, Conus G, Asadi N, Kayal M (2017) Towards the next generation of intelligent building: an assessment study of current automation and future IoT based systems with a proposal for transitional design. Sustain Cities Soc 28:473–481
Mazhar T, Malik MA, Haq I, Rozeela I, Ullah I, Khan MA, Adhikari D, Ben Othman MT, Hamam H (2022) The role of ML, AI, and 5G technology in smart energy and smart building management. Electronics 11:3960
Mohammadi M, Rashid TA, Karim SHT, Aldalwie AHM, Tho QT, Bidaki M, Rahmani AM, Hosseinzadeh M (2021) A comprehensive survey and taxonomy of the SVM-based intrusion detection systems. J Netw Comput Appl 178:102983
Pawar P, Vittal KP (2019) Design and development of advanced smart energy management system integrated with IoT framework in a smart grid environment. J Energy Stor 25:100846
Peña M, Biscarri F, Personal E, León C (2022) Decision support system to classify and optimize the energy efficiency in smart buildings: a data analytics approach. Sensors 22:1380
Piatek K, Firlit A, Chmielowiec K, Dutka M, Barczentewicz S, Hanzelka Z (2021) Optimal selection of metering points for power quality measurements in distribution system. Energies 14:1202
Pong PWT, Annaswamy AM, Kroposki B, Zhang Y, Rajagopal R, Zussman G, Poor HV (2021) Cyber-enabled grids: shaping future energy systems. Adv Appl Energy 1:100003
Raghul M, Jeevitha S, Deveswaran S (2022) Monitoring maximum power point of photovoltaic systems. Int Res J Mod Eng Technol Sci 4:8
Rao CK, Sahoo SK, Balamurugan M, Satapathy SR, Patnaik A, Yanine FF (2020) Applications of sensors in solar energy systems. In 2020 international conference on renewable energy integration into smart grids: a multidisciplinary approach to technology modelling and simulation (ICREISG). IEEE
Rao CK, Sahoo SK, Yanine FF (2021a) Demand response for renewable generation in an IoT based intelligent smart energy management system. In: 2021 innovations in power and advanced computing technologies (i-PACT), Kuala Lumpur, Malaysia. pp 1–7
Rao CK, Sahoo SK, Balamurugan M, Yanine FF (2021b) Design of smart socket for monitoring of IoT-based intelligent smart energy management system. In: Lecture notes in electrical engineering. Springer, Singapore, pp 503–518. https://doi.org/10.1007/978-981-15-8439-8_41
Rao CK, Sahoo SK, Yanine FF (2022) Forecasting electric power generation in photovoltaic power systems for smart energy management. In: 2022 international conference on intelligent controller and computing for smart power (ICICCSP)
Rao CK, Sahoo SK, Yanine FF (2023) A literature review on an IoT-based intelligent smart energy management systems for PV power generation. In: Blanco I (ed) Hybrid advances. Elsevier BV, p 100136. https://doi.org/10.1016/j.hybadv.2023.100136
Rao CK, Sahoo SK, Yanine FF (2024) Demand side energy management algorithms integrated with the IoT framework in the PV smart grid system. In: Arya Y (ed) Advanced frequency regulation strategies in renewable-dominated power systems. Elsevier, pp 255–277
Raza M, Barket AR, Rehman AU, Rehman A, Ullah I (2020) Mobile crowdsensing based architecture for intelligent traffic prediction and quickest path selection. In: Proceedings of the 2020 international conference on UK–China emerging technologies (UCET), Glasgow, UK, 20–21 August 2020. pp 1–4
Rehman AU, Wadud Z, Elavarasan RM, Hafeez G, Khan I, Shafiq Z, Alhelou HH (2021) An optimal power usage scheduling in a smart grid integrated with renewable energy sources for energy management. IEEE Access 9:84619–84638
Sarker IH, Colman A, Han J, Khan AI, Abushark YB, Salah K (2020) Behavdt: A behavioral decision tree learning to build a user-centric context-aware predictive model. Mob Netw Appl 25:1151–1161
Shah SFA, Iqbal M, Aziz Z, Rana TA, Khalid A, Cheah YN, Arif M (2022) The role of machine learning and the Internet of things in smart buildings for energy efficiency. Appl Sci 12:7882
Shajin FH, Rajesh P (2020) Trusted secure geographic routing protocol: outsider attack detection in mobile ad hoc networks by adopting trusted secure geographic routing protocol. Int J Pervasive Comput Commun 18(5):603–621
Xiaoyi Z, Dongling W, Yuming Z, Manokaran KB, Antony AB (2021) IoT-drive framework-based efficient green energy management in smart cities using multi-objective distributed dispatching algorithm Environ. Impact Assess Rev 88:106567
Yousafzai BK, Khan SA, Rahman T, Khan I, Ullah I, Ur Rehman A, Baz M, Hamam H, Cheikhrouhou O (2021) Student-performulator: Student academic performance using hybrid deep neural network. Sustainability 13:9775
Yu L (2020) Deep reinforcement learning for smart building energy management: a survey. arXiv:2008.05074
Zhang D, Han X, Deng C (2018) Review on the research and practice of deep learning and reinforcement learning in smart grids. CSEE J Power Energy Syst 4:362–370
Zhang H, Feng H, Hewage K, Arashpour M (2022) Artificial neural network for predicting building energy performance: a surrogate energy retrofits decision support framework. Buildings 12:829
Download references
Acknowledgements
Not applicable.
The author(s) declare that no financial support was received for the research, authorship, and/or publication of this article.
Author information
Authors and affiliations.
Department of Electrical Engineering, Parala Maharaja Engineering College, Berhampur, affiliated to Biju Patnaik University of Technology, Rourkela, Odisha, India
Challa Krishna Rao & Sarat Kumar Sahoo
Department of Electrical and Electronics Engineering, Aditya Institute of Technology and Management, Tekkali, Andhra Pradesh, India
Challa Krishna Rao
Faculty of Engineering, Universidad Finis Terrae, Providencia, Santiago, Chile
Franco Fernando Yanine
You can also search for this author in PubMed Google Scholar
Contributions
CKR: Conceptualization, Formal Analysis, Investigation, Methodology, Supervision, Validation, Writing – original draft. Prof. SKS: Conceptualization, Formal Analysis, Investigation, Methodology, Supervision, Validation, Writing – original draft. FFY: Conceptualization, Data curtain, Formal Analysis, Investigation, Methodology, Software, Validation, Writing – original draft.
Corresponding author
Correspondence to Challa Krishna Rao .
Ethics declarations
Competing interest.
The authors declare that the research was conducted in the absence of any commercial or financial relationships that could be construed as a potential conflict of interest.
Additional information
Publisher's note.
Springer Nature remains neutral with regard to jurisdictional claims in published maps and institutional affiliations.
Rights and permissions
Springer Nature or its licensor (e.g. a society or other partner) holds exclusive rights to this article under a publishing agreement with the author(s) or other rightsholder(s); author self-archiving of the accepted manuscript version of this article is solely governed by the terms of such publishing agreement and applicable law.
Reprints and permissions
About this article
Rao, C.K., Sahoo, S.K. & Yanine, F.F. An IoT Enabled Energy Management System with Precise Forecasting and Load Optimization for PV Power Generation. Trans Indian Natl. Acad. Eng. (2024). https://doi.org/10.1007/s41403-024-00498-z
Download citation
Received : 13 March 2024
Accepted : 25 July 2024
Published : 27 August 2024
DOI : https://doi.org/10.1007/s41403-024-00498-z
Share this article
Anyone you share the following link with will be able to read this content:
Sorry, a shareable link is not currently available for this article.
Provided by the Springer Nature SharedIt content-sharing initiative
- Renewable generation
- Energy consumption
- Load modeling
- Smart grids
- Demand-side energy management
- Machine learning
- Energy management systems
- Find a journal
- Publish with us
- Track your research
- Today's news
- Reviews and deals
- Climate change
- 2024 election
- Fall allergies
- Health news
- Mental health
- Sexual health
- Family health
- So mini ways
- Unapologetically
- Buying guides
Entertainment
- How to Watch
- My Portfolio
- Latest News
- Stock Market
- Biden Economy
- Stocks: Most Actives
- Stocks: Gainers
- Stocks: Losers
- Trending Tickers
- World Indices
- US Treasury Bonds Rates
- Top Mutual Funds
- Options: Highest Open Interest
- Options: Highest Implied Volatility
- Basic Materials
- Communication Services
- Consumer Cyclical
- Consumer Defensive
- Financial Services
- Industrials
- Real Estate
- Stock Comparison
- Advanced Chart
- Currency Converter
- Credit Cards
- Balance Transfer Cards
- Cash-back Cards
- Rewards Cards
- Travel Cards
- Credit Card Offers
- Best Free Checking
- Student Loans
- Personal Loans
- Car insurance
- Mortgage Refinancing
- Mortgage Calculator
- Morning Brief
- Market Domination
- Market Domination Overtime
- Asking for a Trend
- Opening Bid
- Stocks in Translation
- Lead This Way
- Good Buy or Goodbye?
- Financial Freestyle
- Capitol Gains
- Fantasy football
- Pro Pick 'Em
- College Pick 'Em
- Fantasy baseball
- Fantasy hockey
- Fantasy basketball
- Download the app
- Daily fantasy
- Scores and schedules
- GameChannel
- World Baseball Classic
- Premier League
- CONCACAF League
- Champions League
- Motorsports
- Horse racing
- Newsletters
New on Yahoo
- Privacy Dashboard
Yahoo Finance
Renewables accounted for 14.6% of global energy consumption in 2023.
In June, the Energy Institute released the 2024 Statistical Review of World Energy . The Review provides a comprehensive picture of supply and demand for major energy sources on a country-level basis. Each year, I write a series of articles covering the Review’s findings.
In previous articles, I discussed:
Overall highlights
Trends in global carbon dioxide emissions
Global production and consumption of petroleum
Global production and consumption of natural gas
Global production and consumption of coal
Trends in nuclear power
Today I will discuss renewable energy, with a focus on the growth of wind and solar power.
In 2023, renewable energy sources surged to new heights. Renewables’ share of total primary energy consumption reached 14.6%, 0.4% above the previous year.
Solar and wind power drove global renewable electricity generation to a record-breaking 4,748 TWh, marking a 13% increase from the previous year. This growth accounted for 74% of all net additional electricity generated worldwide.
Solar power led the charge, with 346 GW of new capacity, smashing the 2022 record by 67%. China contributed a quarter of this growth. Europe, too, made significant strides, adding over 56 GW of solar capacity, making up 16% of the global total capacity increase.
Global Renewable Consumption (excluding hydropower). Robert Rapier
Wind power also soared to new heights, with over 115 GW of new capacity installed—another record. China again was at the forefront, responsible for nearly 66% of these additions. China’s total installed wind capacity now rivals that of North America and Europe combined. Offshore wind, a growing frontier in renewable energy, saw Europe holding the highest share at 12%, but China wasn’t far behind, boasting 37 GW compared to Europe’s 32 GW.
Meanwhile, the share of biofuels increased in the global energy mix. Production grew by over 17% from 2022, with the United States and Brazil leading the way. In 2024, bio-gasoline (predominantly ethanol) and biodiesel production reached a near-even split, with the U.S., Brazil, and Europe consuming the lion’s share of these renewable fuels.
The Top Producers
China dominates the world’s renewable energy production. Notably, both China and India — which have seen dramatic fossil fuel consumption growth in recent years — have increased renewable consumption at double-digit rates over the past decade.
Top 10 Renewable Energy Producers in 2023. Robert Rapier
There are a couple of caveats to note about this table. First, it excludes hydropower. The reason is even though hydropower generation contributes around as much as wind and solar, hydropower growth has been relatively stable for years. This table basically shows the growth trajectory of modern renewables like wind and solar power.
Second, the numbers are reported as “Input-equivalent energy”, which is the amount of fuel that would be required by thermal power. This accounts for the lower efficiencies of converting coal, for example, into electricity. In other words, for a given amount of solar power, the table is calculating how much coal or natural gas would be required to produce that much power.
Conclusions
Renewable energy, particularly wind and solar, continue to grow at rapid rates. With record-breaking growth in capacity and generation, these modern renewables continue to supplement traditional energy sources.
China continues to dominate the renewable sector, driving much of the global expansion, while the U.S., Europe, and Brazil also make significant contributions, particularly in biofuels.
As the world strives to reduce carbon emissions and transition to cleaner energy, renewables will play a critical role in shaping a sustainable and resilient energy future. However, to date overall energy demand continues to outpace the growth in renewables, which has meant that fossil fuel consumption has also continued to grow.
By Robert Rapier
More Top Reads From Oilprice.com
Looming Atlantic Current Collapse Threatens Northern Europe's Climate
Oil Dominates the $5 Trillion Global Commodity Market
Azerbaijan Aims to Become Key Trade and Manufacturing Hub for China
Read this article on OilPrice.com
Information
- Author Services
Initiatives
You are accessing a machine-readable page. In order to be human-readable, please install an RSS reader.
All articles published by MDPI are made immediately available worldwide under an open access license. No special permission is required to reuse all or part of the article published by MDPI, including figures and tables. For articles published under an open access Creative Common CC BY license, any part of the article may be reused without permission provided that the original article is clearly cited. For more information, please refer to https://www.mdpi.com/openaccess .
Feature papers represent the most advanced research with significant potential for high impact in the field. A Feature Paper should be a substantial original Article that involves several techniques or approaches, provides an outlook for future research directions and describes possible research applications.
Feature papers are submitted upon individual invitation or recommendation by the scientific editors and must receive positive feedback from the reviewers.
Editor’s Choice articles are based on recommendations by the scientific editors of MDPI journals from around the world. Editors select a small number of articles recently published in the journal that they believe will be particularly interesting to readers, or important in the respective research area. The aim is to provide a snapshot of some of the most exciting work published in the various research areas of the journal.
Original Submission Date Received: .
- Active Journals
- Find a Journal
- Proceedings Series
- For Authors
- For Reviewers
- For Editors
- For Librarians
- For Publishers
- For Societies
- For Conference Organizers
- Open Access Policy
- Institutional Open Access Program
- Special Issues Guidelines
- Editorial Process
- Research and Publication Ethics
- Article Processing Charges
- Testimonials
- Preprints.org
- SciProfiles
- Encyclopedia
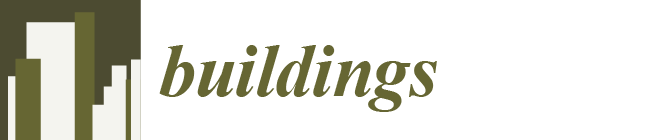
Article Menu
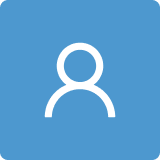
- Subscribe SciFeed
- Recommended Articles
- Google Scholar
- on Google Scholar
- Table of Contents
Find support for a specific problem in the support section of our website.
Please let us know what you think of our products and services.
Visit our dedicated information section to learn more about MDPI.
JSmol Viewer
Renewable energy technology selection for hotel buildings: a systematic approach based on ahp and vikor methods.
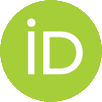
1. Introduction
2. materials and methods, 2.1. literature review, 2.1.1. renewable energy technologies for hotel buildings, 2.1.2. criteria related to renewable energy technologies, 2.2. expert group interviews, 2.3. renewable energy technology selection: ahp and vikor methods, 2.3.2. vikor, 3. results and discussion, 3.1. analysis of technologies based on criteria, 3.1.1. initial investment cost, 3.1.2. operating and maintenance/repair cost, 3.1.3. payback period, 3.1.4. energy production capacity, 3.1.5. maintenance ease and simple management, 3.1.6. reliability, 3.1.7. noise level [ 25 ], 3.1.8. natural resource utilization, 3.1.9. carbon footprint, 3.2. renewable energy technologies, 3.2.1. photovoltaic panels, 3.2.2. solar thermal panels, 3.2.3. solar-powered absorption chiller, 3.2.4. heat pumps, 3.2.5. micro-hydropower, 3.2.6. small-scale wind turbines, 3.2.7. biomass boilers, 3.2.8. integrated systems (photovoltaic panels + heat pumps), 4. conclusions, author contributions, institutional review board statement, data availability statement, acknowledgments, conflicts of interest.
A1 | A2 | A3 | A4 | A5 | A6 | A7 | A8 | |
---|---|---|---|---|---|---|---|---|
A1 | 1 | 1 | 3 | 0.2 | 0.33 | 0.33 | 0.33 | 3 |
A2 | 1 | 1 | 3 | 0.2 | 0.33 | 0.33 | 0.33 | 3 |
A3 | 0.33 | 0.33 | 1 | 0.14 | 0.2 | 0.2 | 0.2 | 1 |
A4 | 5 | 5 | 7 | 1 | 3 | 3 | 3 | 7 |
A5 | 3 | 3 | 5 | 0.33 | 1 | 1 | 1 | 7 |
A6 | 3 | 3 | 5 | 0.33 | 1 | 1 | 1 | 5 |
A7 | 3 | 3 | 5 | 0.33 | 1 | 1 | 1 | 3 |
A8 | 0.33 | 0.33 | 1 | 0.14 | 0.14 | 0.2 | 0.33 | 1 |
Total | 16.66 | 16.66 | 30 | 2.67 | 7 | 7.06 | 7.19 | 30 |
CR = 0.03 |
A1 | A2 | A3 | A4 | A5 | A6 | A7 | A8 | |
---|---|---|---|---|---|---|---|---|
A1 | 1 | 1 | 5 | 3 | 3 | 5 | 5 | 3 |
A2 | 1 | 1 | 5 | 3 | 3 | 5 | 5 | 3 |
A3 | 0.2 | 0.2 | 1 | 0.33 | 0.33 | 1 | 1 | 1 |
A4 | 0.33 | 0.33 | 3 | 1 | 1 | 3 | 3 | 1 |
A5 | 0.33 | 0.33 | 3 | 1 | 1 | 3 | 3 | 1 |
A6 | 0.2 | 0.2 | 1 | 0.33 | 0.33 | 1 | 1 | 0.33 |
A7 | 0.2 | 0.2 | 1 | 0.33 | 0.33 | 1 | 1 | 0.33 |
A8 | 0.33 | 0.33 | 1 | 1 | 1 | 3 | 3 | 1 |
Total | 3.590 | 3.590 | 20.000 | 9.990 | 9.990 | 22.000 | 22.000 | 10.660 |
CR = 0.02 |
A1 | A2 | A3 | A4 | A5 | A6 | A7 | A8 | |
---|---|---|---|---|---|---|---|---|
A1 | 1 | 0.33 | 3 | 1 | 5 | 5 | 1 | 3 |
A2 | 3 | 1 | 5 | 3 | 7 | 7 | 3 | 5 |
A3 | 0.33 | 0.2 | 1 | 0.33 | 3 | 3 | 0.33 | 1 |
A4 | 1 | 0.33 | 3 | 1 | 5 | 5 | 1 | 3 |
A5 | 0.2 | 0.14 | 0.33 | 0.2 | 1 | 1 | 0.2 | 0.33 |
A6 | 0.2 | 0.14 | 0.33 | 0.2 | 1 | 1 | 0.2 | 0.33 |
A7 | 1 | 0.33 | 3 | 1 | 5 | 5 | 1 | 3 |
A8 | 0.33 | 0.2 | 1 | 0.33 | 3 | 3 | 0.33 | 1 |
Total | 7.060 | 2.670 | 16.660 | 7.060 | 30.000 | 30.000 | 7.060 | 16.660 |
CR = 0.03 |
A1 | A2 | A3 | A4 | A5 | A6 | A7 | A8 | |
---|---|---|---|---|---|---|---|---|
A1 | 1 | 3 | 5 | 7 | 5 | 7 | 7 | 1 |
A2 | 0.33 | 1 | 3 | 5 | 3 | 5 | 5 | 0.33 |
A3 | 0.2 | 0.33 | 1 | 3 | 1 | 3 | 3 | 0.2 |
A4 | 0.14 | 0.2 | 0.33 | 1 | 0.33 | 1 | 1 | 0.14 |
A5 | 0.2 | 0.33 | 1 | 3 | 1 | 1 | 3 | 0.2 |
A6 | 0.14 | 0.2 | 0.33 | 1 | 1 | 1 | 1 | 0.14 |
A7 | 0.14 | 0.2 | 0.33 | 1 | 0.33 | 1 | 1 | 0.14 |
A8 | 1 | 3 | 5 | 7 | 5 | 7 | 7 | 1 |
Total | 3.150 | 8.260 | 15.990 | 28.000 | 16.660 | 26.000 | 28.000 | 3.150 |
CR = 0.04 |
A1 | A2 | A3 | A4 | A5 | A6 | A7 | A8 | |
---|---|---|---|---|---|---|---|---|
A1 | 1 | 1 | 3 | 3 | 3 | 3 | 5 | 3 |
A2 | 1 | 1 | 3 | 3 | 3 | 3 | 5 | 3 |
A3 | 0.33 | 0.33 | 1 | 1 | 1 | 1 | 3 | 1 |
A4 | 0.33 | 0.33 | 1 | 1 | 1 | 1 | 3 | 1 |
A5 | 0.33 | 0.33 | 1 | 1 | 1 | 1 | 3 | 1 |
A6 | 0.33 | 0.33 | 1 | 1 | 1 | 1 | 3 | 1 |
A7 | 0.2 | 0.2 | 0.33 | 0.33 | 0.33 | 0.33 | 1 | 0.33 |
A8 | 0.33 | 0.33 | 1 | 1 | 1 | 1 | 3 | 1 |
Total | 3.850 | 3.850 | 11.330 | 11.330 | 11.330 | 11.330 | 26.000 | 11.330 |
CR = 0.01 |
A1 | A2 | A3 | A4 | A5 | A6 | A7 | A8 | |
---|---|---|---|---|---|---|---|---|
A1 | 1 | 1 | 3 | 1 | 1 | 3 | 5 | 3 |
A2 | 1 | 1 | 3 | 1 | 1 | 3 | 5 | 3 |
A3 | 0.33 | 0.33 | 1 | 0.33 | 0.33 | 1 | 3 | 1 |
A4 | 1 | 1 | 3 | 1 | 1 | 3 | 5 | 3 |
A5 | 1 | 1 | 3 | 1 | 1 | 3 | 5 | 3 |
A6 | 0.33 | 0.33 | 1 | 0.33 | 0.33 | 1 | 3 | 1 |
A7 | 0.2 | 0.2 | 0.33 | 0.2 | 0.2 | 0.33 | 1 | 0.33 |
A8 | 0.33 | 0.33 | 1 | 0.33 | 0.33 | 1 | 3 | 1 |
Total | 5.190 | 5.190 | 15.330 | 5.190 | 5.190 | 15.330 | 30.000 | 15.330 |
CR = 0.01 |
A1 | A2 | A3 | A4 | A5 | A6 | A7 | A8 | |
---|---|---|---|---|---|---|---|---|
A1 | 1 | 1 | 1 | 3 | 5 | 7 | 5 | 3 |
A2 | 1 | 1 | 1 | 3 | 5 | 7 | 5 | 3 |
A3 | 1 | 1 | 1 | 3 | 5 | 7 | 5 | 3 |
A4 | 0.33 | 0.33 | 0.33 | 1 | 3 | 5 | 3 | 1 |
A5 | 0.2 | 0.2 | 0.2 | 0.33 | 1 | 3 | 1 | 0.33 |
A6 | 0.14 | 0.14 | 0.14 | 0.2 | 0.33 | 1 | 0.33 | 0.2 |
A7 | 0.2 | 0.2 | 0.2 | 0.33 | 1 | 3 | 1 | 0.33 |
A8 | 0.33 | 0.33 | 0.33 | 1 | 3 | 5 | 3 | 1 |
Total | 4.200 | 4.200 | 4.200 | 11.860 | 23.330 | 38.000 | 23.330 | 11.860 |
CR = 0.01 |
A1 | A2 | A3 | A4 | A5 | A6 | A7 | A8 | |
---|---|---|---|---|---|---|---|---|
A1 | 1 | 1 | 1 | 3 | 3 | 1 | 5 | 3 |
A2 | 1 | 1 | 1 | 3 | 3 | 1 | 5 | 3 |
A3 | 1 | 1 | 1 | 3 | 3 | 1 | 5 | 3 |
A4 | 0.33 | 0.33 | 0.33 | 1 | 1 | 0.33 | 3 | 1 |
A5 | 0.33 | 0.33 | 0.33 | 1 | 1 | 0.33 | 3 | 1 |
A6 | 1 | 1 | 1 | 3 | 3 | 1 | 5 | 3 |
A7 | 0.2 | 0.2 | 0.2 | 0.33 | 0.33 | 0.2 | 1 | 0.33 |
A8 | 0.33 | 0.33 | 0.33 | 1 | 1 | 0.33 | 3 | 1 |
Total | 5.190 | 5.190 | 5.190 | 15.330 | 15.330 | 5.190 | 30.000 | 15.330 |
CR = 0.01 |
A1 | A2 | A3 | A4 | A5 | A6 | A7 | A8 | |
---|---|---|---|---|---|---|---|---|
A1 | 1 | 1 | 1 | 3 | 1 | 1 | 5 | 3 |
A2 | 1 | 1 | 1 | 3 | 1 | 1 | 5 | 3 |
A3 | 1 | 1 | 1 | 3 | 1 | 1 | 5 | 3 |
A4 | 0.33 | 0.33 | 0.33 | 1 | 0.33 | 0.33 | 3 | 1 |
A5 | 1 | 1 | 1 | 3 | 1 | 1 | 5 | 3 |
A6 | 1 | 1 | 1 | 3 | 1 | 1 | 5 | 3 |
A7 | 0.2 | 0.2 | 0.2 | 0.33 | 0.2 | 0.2 | 1 | 0.33 |
A8 | 0.33 | 0.33 | 0.33 | 1 | 0.33 | 0.33 | 3 | 1 |
Total | 5.860 | 5.860 | 5.860 | 17.330 | 5.860 | 5.860 | 32.000 | 17.330 |
CR = 0.01 |
Number of criteria | |
The largest eigenvalue of the decision matrix | |
Random consistency index | |
Consistency Index | |
Consistency Ratio | |
Utility measures for alternative j | |
Regret measures for alternative j | |
VIKOR ranking index for alternative j | |
Weight of criterion i | |
Best (ideal) value for criterion i | |
Worst (anti-ideal) value for criterion i | |
Value of criterion i for alternative j | |
Summation operation | |
Minimum value | |
Maximum value | |
Minimum value | |
Maximum value | |
Weight given to the maximum group utility by the decision-maker ( ) | |
VIKOR ranking index for the best alternative | |
VIKOR ranking index for the best alternative | |
Number of alternatives |
- European Parliament and Council. Directive (EU) 2023/2413 of the European Parliament and of the Council of 18 October 2023 Amending Directive (EU) 2018/2001, Regulation (EU) 2018/1999 and Directive 98/70/EC as Regards the Promotion of Energy from Renewable Sources, and Repealing Council Directive (EU) 2015/652; PE/36/2023/REV/2. Available online: https://eur-lex.europa.eu/legal-content/EN/TXT/?uri=CELEX%3A32023L2413 (accessed on 10 May 2024).
- United Nations. Paris Agreement. Available online: https://unfccc.int/process-and-meetings/the-paris-agreement (accessed on 8 March 2024).
- Karapidakis, E.; Markaki, M.; Kikaki, A.; Yfanti, S.; Nikologiannis, M. Introduction of Electricity Storage and Photovoltaics for an Adequate Self-Sufficiency in Large Building Complexes. WSEAS Trans. Environ. Dev. 2024 , 20 , 37–45. [ Google Scholar ] [ CrossRef ]
- Tourism UN Environment Programme. UN Environment Programme. Tourism 2022. Available online: https://www.unep.org/explore-topics/resource-efficiency/what-we-do/responsible-industry/tourism (accessed on 10 May 2024).
- Luo, J.; Mastani Joybari, M.; Ma, Y.; Liu, J.; Lai, K.-H. Assessment of Renewable Power Generation Applied in Homestay Hotels: Energy and Cost-Benefit Considering Dynamic Occupancy Rates and Reservation Prices. J. Build. Eng. 2024 , 87 , 109074. [ Google Scholar ] [ CrossRef ]
- Dwyer, L. Resident Well-Being and Sustainable Tourism Development: The ‘Capitals Approach’. J. Sustain. Tour. 2023 , 31 , 2119–2135. [ Google Scholar ] [ CrossRef ]
- Pace, L.A. How Do Tourism Firms Innovate for Sustainable Energy Consumption? A Capabilities Perspective on the Adoption of Energy Efficiency in Tourism Accommodation Establishments. J. Clean. Prod. 2016 , 111 , 409–420. [ Google Scholar ] [ CrossRef ]
- Karapidakis, E.; Nikologiannis, M.; Markaki, M.; Kikaki, A.; Yfanti, S. Assessment of Batteries’ Contribution for Optimal Self-Sufficiency in Large Building Complexes. Appl. Syst. Innov. 2023 , 6 , 107. [ Google Scholar ] [ CrossRef ]
- D’Amore, L.; Kalifungwa, P. Meeting the Challenges of Climate Change to Tourism: Case Studies of Best Practice ; Cambridge Scholars Publishing: Newcastle upon Tyne, UK, 2013. [ Google Scholar ]
- Chauhan, A.; Saini, R.P. A review on Integrated Renewable Energy System based power generation for stand-alone applications: Configurations, storage options, sizing methodologies and control. Renew. Sustain. Energy Rev. 2014 , 38 , 99–120, ISSN 1364-0321. [ Google Scholar ] [ CrossRef ]
- Gonçalves, P.; Rodrigues Gaspar, A.; Gameiro da Silva, M. Energy and Exergy-Based Indicators for the Energy Performance Assessment of a Hotel Building. Energy Build. 2012 , 52 , 181–188. [ Google Scholar ] [ CrossRef ]
- Mardani, A.; Zavadskas, E.K.; Streimikiene, D.; Jusoh, A.; Nor, K.M.D.; Khoshnoudi, M. Using fuzzy multiple criteria decision making approaches for evaluating energy saving technologies and solutions in five star hotels: A new hierarchical framework. Energy 2016 , 117 , 131–148. [ Google Scholar ] [ CrossRef ]
- Iturralde Carrera, L.; Ramírez Ceballos, J.C.; Molina-Santana, E.; Castillo, F.; Cruz-Fernández, M.; Álvarez-Alvarado, J.; Rodriguez, J. Integration of Energy Management and Efficiency System for Buildings With Zero Carbon Emissions: A Case of Study. IEEE Access 2024 , 12 , 64237–64251. [ Google Scholar ] [ CrossRef ]
- Abdou, A.H.; Hassan, T.H.; El Dief, M.M. A Description of Green Hotel Practices and Their Role in Achieving Sustainable Development. Sustainability 2020 , 12 , 9624. [ Google Scholar ] [ CrossRef ]
- Abdelhady, S. Techno-Economic Study and the Optimal Hybrid Renewable Energy System Design for a Hotel Building with Net Zero Energy and Net Zero Carbon Emissions. Energy Convers. Manag. 2023 , 289 , 117195. [ Google Scholar ] [ CrossRef ]
- Han, H.; Yoon, H. Customer retention in the eco-friendly hotel sector: Examining the diverse processes of post-purchase decision-making. J. Sustain. Tour. 2015 , 23 , 1095–1113. [ Google Scholar ] [ CrossRef ]
- Hou, H.; Wu, H. Tourists’ perceptions of green building design and their intention of staying in green hotel. Tour. Hosp. Res. 2020 , 21 , 115–128. [ Google Scholar ] [ CrossRef ]
- Trišić, I.; Štetić, S.; Privitera, D.; Petrović, M.D.; Maksin, M.; Vujović, S.; Jovanović, Z.; Kalinić, M. Perspectives on Sustainable Tourism Development in the Hotel Industry—A Case Study from Southern Europe. Sustainability 2021 , 13 , 5563. [ Google Scholar ] [ CrossRef ]
- Abdilahi, A.M.; Yatim, A.H.M.; Mustafa, M.W.; Khalaf, O.T.; Shumran, A.F.; Nor, F.M. Feasibility study of renewable energy-based microgrid system in Somaliland’s urban centers. Renew. Sustain. Energy Rev. 2014 , 40 , 1048–1059. [ Google Scholar ] [ CrossRef ]
- Yazdani-Chamzini, A.; Fouladgar, M.M.; Zavadskas, E.K.; Moini, S.H.H. Selecting the optimal renewable energy using multi criteria decision making. J. Bus. Econ. Manag. 2013 , 14 , 957–978. [ Google Scholar ] [ CrossRef ]
- Sreeraj, E.S.; Chatterjee, K.; Bandyopadhyay, S. Design of Isolated Renewable Hybrid Power Systems. Sol. Energy 2010 , 84 , 1124–1136. [ Google Scholar ] [ CrossRef ]
- Bajpai, P.; Dash, V. Hybrid renewable energy systems for power generation in stand-alone applications: A review. Renew. Sustain. Energy Rev. 2012 , 16 , 2926–2939. [ Google Scholar ] [ CrossRef ]
- Li, Y.; Kumar, G.M.S.; Cao, S.; Wang, S. Electric vehicle-based distant energy sharing between zero-emission coastal office and hotel buildings. J. Build. Eng. 2024 , 90 , 109496, ISSN 2352-7102. [ Google Scholar ] [ CrossRef ]
- Fadaee, M.; Radzi, M.A.M. Multi-objective optimization of a stand-alone hybrid renewable energy system by using evolutionary algorithms: A review. Renew. Sustain. Energy Rev. 2012 , 16 , 3364–3369. [ Google Scholar ] [ CrossRef ]
- Zheng, C.; Wang, J. Optimization of hybrid PV/wind/battery systems for grid-connected and stand-alone applications under different scenarios. Energy 2020 , 195 , 116973. [ Google Scholar ]
- Güğül, G.N. Optimum hybrid renewable energy system design for on and off grid buildings: Hotel, education and animal hospital building case. Sol. Energy 2023 , 253 , 414–427. [ Google Scholar ] [ CrossRef ]
- Luo, X.; Liu, H.; Li, H.; Wei, H.; Zhou, G. Techno-economic feasibility of a hybrid wind-wave energy system for zero-energy coastal hotel buildings. Energy Rep. 2022 , 8 , 2101–2115. [ Google Scholar ] [ CrossRef ]
- Navratil, J.; Navratil, P.; Geletka, V.; Suchacek, P.; Vochozka, M. Analysis of the potential and current utilization of renewable energy sources in various types of buildings in the Czech Republic. Renew. Energy 2019 , 139 , 1283–1297. [ Google Scholar ]
- Niveditha, N.; Singaravel, M.R. Optimal sizing of hybrid PV–Wind–Battery storage system for Net Zero Energy Buildings to reduce grid burden. Appl. Energy 2022 , 324 , 119713. [ Google Scholar ] [ CrossRef ]
- Sami, S.; Gholizadeh, M.; Dadpour, D.; Deymi-Dashtebayaz, M. Design and optimization of a CCHDP system integrated with NZEB from energy, exergy and exergoeconomic perspectives. Energy Convers. Manag. 2022 , 271 , 116347. [ Google Scholar ] [ CrossRef ]
- Żołądek, M.; Figaj, R.; Kafetzis, A.; Panopoulos, K. Energy-economic assessment of self-sufficient microgrid based on wind turbine, photovoltaic field, wood gasifier, battery, and hydrogen energy storage. Int. J. Hydrogen Energy 2024 , 52 , 728–744. [ Google Scholar ] [ CrossRef ]
- Zhou, S.; Cao, S.; Wang, S. Realisation of a coastal zero-emission office building with the support of hybrid ocean thermal, floating photovoltaics, and tidal stream generators. Energy Convers. Manag. 2022 , 253 , 115135. [ Google Scholar ] [ CrossRef ]
- Petrevska, B.; Cingoski, V.; Serafimova, M. Sustainable tourism and hotel management in Macedonia through the use of renewable energy sources. UTMS J. Econ. 2016 , 7 , 123–132, ISSN 1857-6974. [ Google Scholar ]
- Cesur, F.; Taş, N.; Taş, M. A Decision-Making Model Proposal for the Use of Renewable Energy Technologies in Buildings in Turkey. Energies 2024 , 17 , 2354. [ Google Scholar ] [ CrossRef ]
- SHURA. Rooftop Solar Energy Potential in Buildings—Financing Models and Policies for the Deployment of Rooftop Solar Energy Systems in Turkey ; Energy Transition Center: Calgary, AB, Canada, 2020; pp. 16–58. Available online: www.shura.org.tr (accessed on 8 March 2023).
- Dalton, G.J.; Lockington, D.A.; Baldock, T.E. Feasibility analysis of renewable energy supply options for a grid-connected large hotel. Renew. Energy 2009 , 34 , 955–964. [ Google Scholar ] [ CrossRef ]
- Şumovschi, A.I.; Pentiuc, R.-D.; Atănăsoae, P. Cost-Benefit Analysis for Renewable Energy Generation in a Hotel-type Building. In Proceedings of the 2023 International Conference on Electromechanical and Energy Systems (SIELMEN), Craiova, Romania, 11–13 October 2023; pp. 1–5. [ Google Scholar ] [ CrossRef ]
- Dhirasasna, N.; Sahin, O. A system dynamics model for renewable energy technology adoption of the hotel sector. Renew. Energy 2021 , 163 , 1994–2007. [ Google Scholar ] [ CrossRef ]
- Dhirasasna, N.; Becken, S.; Sahin, O. A systems approach to examining the drivers and barriers of renewable energy technology adoption in the hotel sector in Queensland, Australia. J. Hosp. Tour. Manag. 2020 , 42 , 153–172. [ Google Scholar ] [ CrossRef ]
- Harker, P.T.; Vargas, L.G. The Theory of Ratio Scale Estimation: Saaty’s Analytic Hierarchy Process. Manag. Sci. 1987 , 33 , 1383–1403. [ Google Scholar ] [ CrossRef ]
- Saaty, T. Theory and Applications of the Analytic Network Process: Decision Making with Benefits, Opportunities, Costs and Risks ; RWS Publications: Pittsburgh, PA, USA, 2005. [ Google Scholar ]
- Saaty, T.L. Decision Making with the Analytic Hierarchy Process. Int. J. Serv. Sci. 2008 , 1 , 83–98. [ Google Scholar ] [ CrossRef ]
- Saaty, T.L. The Analytic Hierarchy Process: Planning, Priority Setting, Resource Allocation ; University of Pittsburgh: Pittsburgh, PA, USA, 1988. [ Google Scholar ]
- Arıkan Kargı, V.S. Decision Making In CNC Vertical Machining Center: An Integrated Approach with AHP and COPRAS Methods. In International Studies in Economics and Administrative Sciences ; Serüven: Ankara, Turkey, 2024; pp. 123–137. [ Google Scholar ]
- Opricovic, S.; Tzeng, G.H. Compromise Solution by MCDM Methods: A Comparative Analysis of VIKOR and TOPSIS. Eur. J. Oper. Res. 2004 , 156 , 445–455. [ Google Scholar ] [ CrossRef ]
- Ju, Y.; Wang, A. Extension of VIKOR Method for Multi Criteria Group Decision Making Problem With Linguistic Information. Appl. Math. Modell. 2013 , 37 , 3112–3125. [ Google Scholar ] [ CrossRef ]
- Yu, P.L. A Class of Solutions for Group Decision Problems. Manag. Sci. 1973 , 19 , 936–946. [ Google Scholar ] [ CrossRef ]
- Büyüközkan, G.; Ruan, D. Evaluation of Software Development Projects Using Fuzzy Multi-Criteria Decision Approach. Math. Comput. Simul. 2008 , 77 , 464–475. [ Google Scholar ] [ CrossRef ]
- Opricovic, S.; Tzeng, G.H. Extended VIKOR Method in Comparison with Other Outranking Methods. Eur. J. Oper. Res. 2007 , 178 , 514–529. [ Google Scholar ] [ CrossRef ]
Click here to enlarge figure
Research Steps | Methodology | |
---|---|---|
Qualitative Research | Problem identification | Literature review |
Objectives definition | Expert group interviews | |
Scope determination | Observations | |
Determination of the research method | ||
Research design | ||
Quantitative Research | Determination of RETs | Field study |
Creation of scenarios with different RETs for the hotel building | Field study | |
Determination of the renewable energy technology criteria | Field study | |
Solving the problem of selecting RETs for the hotel building | Multi-criteria decision-making framework | |
Determination of criteria weights | AHP | |
Decision-making | AHP-VIKOR |
Role in RET System | Profession | Experience | Company | |
---|---|---|---|---|
A1 | Designer | Architect | 20 year | Private |
A2 | Designer and builder | Architect | 15 year | Public |
E1 | Designer and builder | Engineer (Mech.) | 20 year | Private |
E2 | Designer and builder | Engineer (Mech.) | 25 year | Public |
E3 | Designer and builder | Engineer (Elec.) | 20 year | Private |
E4 | Designer and builder | Engineer (Elec.) | 15 year | Private |
I1 | Investor | Hotel investor | 30 year | Private |
I2 | Investor | Hotel investor | 25 year | Private |
C1 | Designer and builder | Consultant | 20 year | Private |
C2 | Designer and builder | Consultant | 25 year | Private |
M1 | Designer and builder | RET system manufacturer | 20 year | Private |
M2 | Designer and builder | RET system manufacturer | 25 year | Private |
T1 | Occupant | Hotel maintenance staff | 15 year | Private |
T2 | Occupant | Hotel maintenance staff | 20 year | Private |
Criteria | Description | |
---|---|---|
Economic Criteria | Initial Investment Cost (C1) [ , , , ] | This criterion includes the initial setup and equipment costs of the technology [ , , ]. |
Operating and Maintenance/Repair Cost (C2) [ , , ] | These are the regular maintenance and operational costs of the technologies [ , ]. This includes operating expenses such as the electricity costs required to run the equipment for a year [ ]. | |
Payback Period (C3) [ , ] | This criterion refers to the time it takes for the investment in renewable energy technology to pay for itself through savings and returns [ , ]. | |
Technological Criteria | Energy Production Capacity (C4) [ , ] | Capacity to meet the energy demands of hotel buildings and the ability to provide sufficient power [ , ]. |
Maintenance Ease and Simple Management (C5) | Regular maintenance requirements of the technology, user-friendliness, ease of management, and access to technical support and spare parts. | |
Reliability (C6) [ ] | The technology’s capability to provide a continuous power supply to ensure uninterrupted hotel operations [ ]. | |
Environmental Impact Criteria | Noise Level (C7) [ ] | The potential noise generated by the energy systems and its effects [ ]. |
Natural Resource Utilization (C8) [ ] | The technology’s impact on the consumption of natural resources [ ]. | |
Carbon Footprint (C9) [ ] | The effect of the technology on carbon emissions [ ]. |
Renewable Energy Technologies | Description |
---|---|
Photovoltaic Panels | Solar panels that convert solar energy into electrical energy. |
Solar Thermal Panels | Systems that use solar energy to provide efficiency in water heating systems. |
Solar-Powered Absorption Chiller | Systems that use solar energy for cooling. |
Heat Pumps | Heat pumps that meet the heating and cooling needs of hotel buildings with low energy consumption. |
Micro-Hydropower | Small-scale hydroelectric systems that convert the kinetic energy of water into electrical energy. |
Small-Scale Wind Turbines | Small-scale wind turbines that generate electricity by converting kinetic energy into electrical energy. |
Biomass Boilers | Boilers that produce heat by burning biomass. |
Integrated Systems (Photovoltaic + Heat Pump) [ ] | Systems that integrate photovoltaic panels and heat pumps. |
Steps | Substeps | Description |
---|---|---|
1. Problem Definition and Hierarchy Structure | Problem Definition and Goal | Identification of the decision-making problem and specification of the main objective. |
Criteria Identification | Determination of the criteria required to achieve the goal. | |
Alternatives Identification | Listing of alternatives that will be evaluated under each criterion. | |
Hierarchy Visualization | Creation of a hierarchical structure with the goal at the top, followed by criteria, sub-criteria, and alternatives. | |
2. Pairwise Comparisons and Matrix Construction | Criteria Pairwise Comparison | Evaluation of criteria in pairs to assess their relative importance. |
Saaty’s Scale Application | Use of a scale from 1 to 9 (1: equal importance, 9: extreme importance) to conduct comparisons. | |
Comparison Matrix Construction | Development of a pairwise comparison matrix based on these evaluations. | |
3. Calculation of Weights Using Comparison Matrix | Column Summation | Summation of the values in each column of the comparison matrix. |
Matrix Normalization | Division of each element by the sum of its column to create a normalized matrix. | |
Weights Calculation | Computation of the average of each row in the normalized matrix to determine the weights of the criteria. | |
4. Consistency Ratio Calculation | Consistency Verification | Ensuring that comparisons are consistent by calculating the Consistency Ratio (CR). |
Threshold Compliance | Checking that the CR is below a specified threshold (0.10). | |
Comparison Revision | Reviewing and adjusting pairwise comparisons if the CR exceeds the threshold. | |
5. Pairwise Comparison of Alternatives | Alternatives Evaluation | Identification of the decision-making problem and specification of the main objective. |
Comparison Matrices Development | Determination of the criteria required to achieve the goal. | |
Normalization and Weights Calculation | Listing of alternatives that will be evaluated under each criterion. | |
6. Calculation of Final Scores | Alternatives Weighting | Multiplication of the weights of the alternatives by the criteria weights. |
Score Aggregation | Summation of these products to compute the overall score for each alternative. | |
Alternatives Ranking | Ranking of the alternatives based on their final scores. | |
7. Decision-Making | Best Alternative Selection | Selection of the alternative with the highest score as the optimal solution. |
Relative Importance | Definition | Explanation |
---|---|---|
1 | Equal importance | Two activities contribute equally to objective |
3 | Weak importance | Experience and judgment slightly favor one activity over another |
5 | Strong importance | Experience and judgment strongly favor one activity over another |
7 | Demonstrated importance | One activity is strongly favored and demonstrated in practice |
9 | Extreme importance | The evidence favoring one activity over another is of the highest possible order of affirmation |
2, 4, 6, 8 | İntermediate values | When compromise is needed between two adjacent judgments |
C1 | C2 | C3 | C4 | C5 | C6 | C7 | C8 | C9 | |
---|---|---|---|---|---|---|---|---|---|
1 | 5 | 3 | 3 | 5 | 5 | 5 | 5 | 5 | |
0.2 | 1 | 0.33 | 0.2 | 1 | 0.33 | 1 | 0.33 | 1 | |
0.33 | 3 | 1 | 0.33 | 1 | 1 | 1 | 3 | 3 | |
0.33 | 5 | 3 | 1 | 3 | 3 | 3 | 3 | 3 | |
0.2 | 1 | 1 | 0.33 | 1 | 1 | 3 | 3 | 3 | |
0.2 | 3 | 1 | 0.33 | 1 | 1 | 3 | 5 | 3 | |
0.2 | 1 | 1 | 0.33 | 0.33 | 0.33 | 1 | 3 | 3 | |
0.2 | 3 | 0.33 | 0.33 | 0.33 | 0.2 | 0.33 | 1 | 1 | |
0.2 | 1 | 0.33 | 0.33 | 0.33 | 0.33 | 0.33 | 1 | 1 | |
Total | 2.860 | 23.000 | 10.990 | 6.180 | 12.990 | 12.190 | 17.660 | 24.330 | 23.000 |
C1 | C2 | C3 | C4 | C5 | C6 | C7 | C8 | C9 | |
---|---|---|---|---|---|---|---|---|---|
0.350 | 0.217 | 0.273 | 0.485 | 0.385 | 0.410 | 0.283 | 0.206 | 0.217 | |
0.070 | 0.043 | 0.030 | 0.032 | 0.077 | 0.027 | 0.057 | 0.014 | 0.043 | |
0.115 | 0.130 | 0.091 | 0.053 | 0.077 | 0.082 | 0.057 | 0.123 | 0.130 | |
0.115 | 0.217 | 0.273 | 0.162 | 0.231 | 0.246 | 0.170 | 0.123 | 0.130 | |
0.070 | 0.043 | 0.091 | 0.053 | 0.077 | 0.082 | 0.170 | 0.123 | 0.130 | |
0.070 | 0.130 | 0.091 | 0.053 | 0.077 | 0.082 | 0.170 | 0.206 | 0.130 | |
0.070 | 0.043 | 0.091 | 0.053 | 0.025 | 0.027 | 0.057 | 0.123 | 0.130 | |
0.070 | 0.130 | 0.030 | 0.053 | 0.025 | 0.016 | 0.019 | 0.041 | 0.043 | |
0.070 | 0.043 | 0.030 | 0.053 | 0.025 | 0.027 | 0.019 | 0.041 | 0.043 |
n | 1 | 2 | 3 | 4 | 5 | 6 | 7 | 8 | 9 | 10 | 11 | 12 | 13 | 14 | 15 |
RI | 0.00 | 0.00 | 0.58 | 0.90 | 1.12 | 1.24 | 1.32 | 1.41 | 1.45 | 1.49 | 1.51 | 1.48 | 1.56 | 1.57 | 1.59 |
C1 | C2 | C3 | C4 | C5 | C6 | C7 | C8 | C9 | Final Score | Rank | |
---|---|---|---|---|---|---|---|---|---|---|---|
A1 | 0.021 | 0.012 | 0.015 | 0.055 | 0.024 | 0.021 | 0.016 | 0.009 | 0.007 | 0.179 | 1 |
A2 | 0.021 | 0.012 | 0.032 | 0.029 | 0.024 | 0.021 | 0.016 | 0.009 | 0.007 | 0.171 | 3 |
A3 | 0.010 | 0.002 | 0.006 | 0.014 | 0.008 | 0.008 | 0.016 | 0.009 | 0.007 | 0.080 | 8 |
A4 | 0.106 | 0.005 | 0.015 | 0.006 | 0.008 | 0.021 | 0.007 | 0.003 | 0.002 | 0.175 | 2 |
A5 | 0.051 | 0.005 | 0.003 | 0.013 | 0.008 | 0.021 | 0.003 | 0.003 | 0.007 | 0.115 | 4 |
A6 | 0.049 | 0.002 | 0.003 | 0.007 | 0.008 | 0.008 | 0.002 | 0.009 | 0.007 | 0.094 | 6 |
A7 | 0.046 | 0.002 | 0.015 | 0.006 | 0.003 | 0.004 | 0.003 | 0.002 | 0.001 | 0.082 | 7 |
A8 | 0.010 | 0.004 | 0.006 | 0.055 | 0.008 | 0.008 | 0.007 | 0.003 | 0.002 | 0.105 | 5 |
Steps | Definition |
---|---|
1. Decision Matrix Determination | Matrix Construction Determining Cost and Benefit Criteria Best and Worst Value Determination for Each Criterion Among the Alternatives Normalization of the Decision Matrix Weighting of the Matrix |
2. Utility Measure and Regret Measure Calculation | Calculation ): It reflects the total distance of an alternative from the best value ): It indicates the maximum distance of an alternative from the best value in any criterion |
3. VIKOR Index ( ) Computation | Calculation of Qj Ranking of the Values |
4. Alternative Ranking | Alternative Ranking Values Ranking Rank the alternatives based on the Q values. The alternative with the smallest Q value is considered the best |
5. Condition Satisfaction and Decision-Making | Ensuring Conditions for Selecting the Alternative with the Best Q (min) Value After Ranking Q Values from Smallest to Largest Condition 1: Acceptable benefit condition Condition 2: Acceptable decision reliability Decision-making |
wi | 0.314 | 0.044 | 0.096 | 0.185 | 0.093 | 0.112 | 0.069 | 0.048 | 0.039 |
---|---|---|---|---|---|---|---|---|---|
Min | Min | Min | Max | Max | Max | Min | Min | Min | |
C1 | C2 | C3 | C4 | C5 | C6 | C7 | C8 | C9 | |
A1 | 3 | 3 | 3 | 7 | 5 | 5 | 3 | 3 | 3 |
A2 | 5 | 3 | 1 | 5 | 5 | 5 | 1 | 3 | 1 |
A3 | 3 | 5 | 5 | 3 | 3 | 3 | 5 | 1 | 1 |
A4 | 3 | 3 | 5 | 5 | 3 | 5 | 3 | 3 | 3 |
A5 | 3 | 3 | 7 | 3 | 3 | 5 | 5 | 3 | 1 |
A6 | 3 | 5 | 7 | 1 | 3 | 3 | 7 | 1 | 1 |
A7 | 3 | 5 | 5 | 3 | 1 | 3 | 5 | 5 | 5 |
A8 | 7 | 3 | 5 | 7 | 3 | 3 | 3 | 3 | 3 |
fi* (best value) | 3 | 3 | 1 | 7 | 5 | 5 | 1 | 1 | 1 |
fi- (worst value) | 7 | 5 | 7 | 1 | 1 | 3 | 7 | 5 | 5 |
Sj | Rj | Qj | Sj Ranking | Rj Ranking | Qj Ranking | |
---|---|---|---|---|---|---|
0.099 | 0.032 | 0.000 | 1 | 1 | 1 | |
0.243 | 0.157 | 0.286 | 3 | 4 | 3 | |
0.436 | 0.123 | 0.669 | 5 | 3 | 5 | |
0.239 | 0.064 | 0.278 | 2 | 2 | 2 | |
0.336 | 0.123 | 0.470 | 4 | 3 | 4 | |
0.553 | 0.185 | 0.900 | 6 | 5 | 6 | |
0.569 | 0.123 | 0.933 | 7 | 3 | 7 | |
0.603 | 0.314 | 1.000 | 8 | 6 | 8 |
Qj (v = 0.00) | Qj (v = 0.25) | Qj (v = 0.50) | Qj (v = 0.75) | Qj (v = 1.00) | |
---|---|---|---|---|---|
0.113 | 0.155 | 0.196 | 0.237 | 0.278 | |
) | 0 | 0 | 0 | 0 | 0 |
) | 0.113 | 0.155 | 0.196 | 0.237 | 0.278 |
DQ = 1/(J − 1) | 0.143 | 0.143 | 0.143 | 0.143 | 0.143 |
Condition 1 | Not satisfied | Satisfied | Satisfied | Satisfied | Satisfied |
Condition 2 | Satisfied | Satisfied | Satisfied | Satisfied | Satisfied |
Qj Ranking | Qj (v = 0.00) | Qj Ranking | Qj (v = 0.25) | Qj Ranking | Qj (v = 0.50) | Qj Ranking | Qj (v = 0.75) | Qj Ranking | Qj (v = 1.00) | |
---|---|---|---|---|---|---|---|---|---|---|
1 | 0.000 | 1 | 0.000 | 1 | 0.000 | 1 | 0.000 | 1 | 0.000 | |
4 | 0.443 | 4 | 0.404 | 4 | 0.365 | 3 | 0.325 | 3 | 0.286 | |
3 | 0.324 | 5 | 0.410 | 5 | 0.496 | 5 | 0.582 | 5 | 0.669 | |
2 | 0.113 | 2 | 0.155 | 2 | 0.196 | 2 | 0.237 | 2 | 0.278 | |
3 | 0.324 | 3 | 0.361 | 3 | 0.397 | 4 | 0.434 | 4 | 0.470 | |
5 | 0.543 | 7 | 0.632 | 7 | 0.721 | 7 | 0.811 | 6 | 0.900 | |
3 | 0.324 | 6 | 0.476 | 6 | 0.629 | 6 | 0.781 | 7 | 0.933 | |
6 | 1.000 | 8 | 1.000 | 8 | 1.000 | 8 | 1.000 | 8 | 1.000 |
Renewable Energy Technology Alternatives | VIKOR | AHP |
---|---|---|
Photovoltaic Panels | 1 | 1 |
Solar Thermal Panels | 3 | 3 |
Solar-Powered Absorption Chiller | 5 | 8 |
Heat Pumps | 2 | 2 |
Micro-Hydropower | 4 | 4 |
Small-Scale Wind Turbines | 6 | 6 |
Biomass Boilers | 7 | 7 |
Integrated Systems (Photovoltaic + Heat Pump) | 8 | 5 |
The statements, opinions and data contained in all publications are solely those of the individual author(s) and contributor(s) and not of MDPI and/or the editor(s). MDPI and/or the editor(s) disclaim responsibility for any injury to people or property resulting from any ideas, methods, instructions or products referred to in the content. |
Share and Cite
Arıkan Kargı, V.S.; Cesur, F. Renewable Energy Technology Selection for Hotel Buildings: A Systematic Approach Based on AHP and VIKOR Methods. Buildings 2024 , 14 , 2662. https://doi.org/10.3390/buildings14092662
Arıkan Kargı VS, Cesur F. Renewable Energy Technology Selection for Hotel Buildings: A Systematic Approach Based on AHP and VIKOR Methods. Buildings . 2024; 14(9):2662. https://doi.org/10.3390/buildings14092662
Arıkan Kargı, Vesile Sinem, and Fatma Cesur. 2024. "Renewable Energy Technology Selection for Hotel Buildings: A Systematic Approach Based on AHP and VIKOR Methods" Buildings 14, no. 9: 2662. https://doi.org/10.3390/buildings14092662
Article Metrics
Article access statistics, further information, mdpi initiatives, follow mdpi.
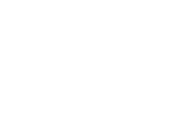
Subscribe to receive issue release notifications and newsletters from MDPI journals

IMAGES
COMMENTS
A review of renewable energy sources, sustainability issues and climate change mitigation ... It is evidential in literature that replacing fossil fuel-based energy sources with renewable energy sources, which includes: bioenergy, direct solar energy, geothermal energy, hydropower, wind and ocean energy (tide and wave), would gradually help the ...
All these are Q1 (energy/renewable energy category) journals apart from Energies and Sustainability which are in the Q2 category while Energy Procedia was discontinued in 2019. 3.4. Renewable energy sources. Fig. 7 is a word cloud generated in python of the different types of renewable energy sources that have been discussed in the publications ...
The use of renewable energy resources, such as solar, wind, and biomass will not diminish their availability. Sunlight being a constant source of energy is used to meet the ever-increasing energy need. This review discusses the world's energy needs, renewable energy technologies for domestic use, and highlights public opinions on renewable energy. A systematic review of the literature was ...
Explore the latest full-text research PDFs, articles, conference papers, preprints and more on ALTERNATIVE ENERGY SOURCES. Find methods information, sources, references or conduct a literature ...
According to IEA's global energy review in 2021, total renewable energy usage has shown a significant increment, from 4,098 TWh in 2010 to 7,627 TWh in 2020. Hydropower contributes the largest portion of renewable energy capacity around the world for electricity generation, even though the growth rate of hydropower is the lowest compared with ...
The transition toward a 100% Renewable Energy System is a complex process with different technical and economic challenges. In order to achieve predetermined goals, several steps should be carried out simultaneously, including increment of energy efficiency, savings in primary energy consumption, and finally, deployment of variable renewable energy sources (VRES) [1].
Heptonstall and Gross systematically review the literature on these costs and asses the range of impacts it is shown to have. The impact of variable renewable energy (VRE) sources on an ...
The role of hydrogen as a clean energy source is a promising but also a contentious issue. The global energy production is currently characterized by an unprecedented shift to renewable energy sources (RES) and their technologies. However, the local and environmental benefits of such RES-based technologies show a wide variety of technological maturity, with a common mismatch to local RES ...
Moreover, these non-renewable resources are available in limited amount, and their formation requires long period. Thus, there is an urgent need to identify the eco-friendly sources of energy which may replace these non-renewable resources and would be able to meet the energy requirements of huge population of the world.
About other renewable energy sources, Turkey had the largest share in generating energy from wind and solar energy, with an annual growth rate of (88.03%, 98.22%), respectively.
Explore the latest full-text research PDFs, articles, conference papers, preprints and more on ALTERNATIVE ENERGY SOURCES. Find methods information, sources, references or conduct a literature ...
Climate change mitigation, the goal of reducing CO2 emissions, more stringent regulations and the increment in energy costs have pushed researchers to study energy efficiency and renewable energy sources. Manufacturing systems are large energy consumers and are thus responsible for huge greenhouse gas emissions; for these reasons, many studies have focused on this topic recently. This review ...
Step by step the energy request is broadening and in this way the necessities for a manageable source that won't hurt the climate are of prime significance. Several projections express that by 2050 the energy requesting will basically increase. ... In this review paper the renewable energy s power and warmth, which is produced using sun based ...
3.1 Renewable Energy Planning and Policy Selecting between alternative energy sources has usually focused only on cost minimization. It is widely recognized now that energy planning is a much more complicated decision with many actors and factors involved. Pohekar and Table 1 Literature Review on MCDM Methods and Application to Renewable Energy ...
Our study highlights the pivotal activities and initiatives driving sustainable energy development. Ultimately, our research reveals that achieving sustainable energy development hinges on three critical actions: transitioning to renewable energy sources, boosting energy efficiency, and mitigating greenhouse gas and air pollutant emissions.
Renewable energy sources hold the key potential to displace greenhouse gas emissions from fossil fuel-based power generating and there-by mitigating climate change (Edenhofer et al., 2011). Sustainable development has become the centre of recent national policies, strategies and devel-opment plans of many countries.
On the supply side, renewable energy sources including bioenergy, ... E. T. Measuring climatic impacts on energy consumption: a review of the empirical literature. Energy Econ. 46, 522-530 (2014).
Renewable energy sources have emerged as an alternative to meet the growing demand for energy, mitigate climate change, and contribute to sustainable development. The integration of these systems is carried out in a distributed manner via microgrid systems; this provides a set of technological solutions that allows information exchange between the consumers and the distributed generation ...
The importance of alternative energy sources comes together with climate change challenges associated with e excessive use of fossil fuels. th primary There are three motivators that stimulate the growth of renewable energy technologies: energy security, economic impacts and carbon dioxide emission reduction. The term "alternative energy"
Wind power, solar power and water power are technologies that can be used as the main sources of renewable energy so that the target of decarbonisation in the energy sector can be achieved. However, when compared with conventional power plants, they have a significant difference. The share of renewable energy has made a difference and posed various challenges, especially in the power ...
The four categories in Table 1, economy, technology, environment, and society, are the main categories that shape the strategical decisions of renewable energy deployment in cities [].The five assessment criteria in Table 1 are extracted from literature [3,4,5,6,7, 11, 18, 34] and belong to one or more of the four categories.Table 1 shows the mapping between the five assessment criteria and ...
In the quest for a sustainable energy landscape, renewable energy sources are positioned to lead. Among these, power purchase agreements (PPAs) have emerged as indispensable tools, particularly when combined with energy storage solutions, in supporting grid stability and ensuring the reliability of energy provision. The aim of this study is to undertake a rigorous systematic literature review ...
VPPs promote the seamless integration of renewable energy sources and provide optimum grid management by aggregating distributed energy resources, which improves sustainability. ... Considering the above research gaps in literature, this review article advances the knowledge of energy systems by providing a thorough analysis of VPPs, their ...
There are various renewable energy resources are available in nature mainly like solar, wind, geo-thermal, tidal, biomass etc. So, in this paper, a brief literature review is carried away to get an idea that how these renewable energy resources had been used so far and to have an idea about the evolution of these energy resources.
The social and political perspectives are important considerations for renewable energy technologies. These perspectives may have impacts that are positive, negative, or a combination. Positive impacts can improve the adoption of certain technologies. Adverse impacts can reduce the intended benefits or even threaten the viability of a ...
The Intelligent Smart Energy Management System (ISEMS) design, depicted in Fig. 1, is tailored for demand-side energy management with a focus on renewable energy sources.Its key components include a predictive smart energy management system, PV generation and data collection, and an Internet of Things (IoT) ecosystem for user information and energy management (Blasi et al. 2022).
Traditional locations for energy generation (e.g. lignite or hard coal mining areas) may lose their substantial significance in favour of renewable energy generation locations, if the former do not possess suitable local conditions for the generation of renewable energy (RE), 1 which leads to economic losses. In contrast, locations where energy ...
In June, the Energy Institute released the 2024 Statistical Review of World Energy. The Review provides a comprehensive picture of supply and demand for major energy sources on a country-level basis.
This study provides an assessment of renewable energy technology utilization in hotel buildings, which are significant structures in terms of energy consumption. The aim of the study is to determine suitable renewable energy technologies (RETs) for hotel buildings by defining criteria for evaluating RETs, assessing the relative importance of these criteria, and proposing a multi-criteria ...